6 Basic Chemistry of Life
The way that atoms behave and interact is defined by their subatomic particles, and in particular the number of electrons in an atom. Here we discuss how and why atoms interact to form molecules as well as how to represent these molecules. We conclude with a deep dive on the elements most commonly found in life.
Learning Objectives
By the end of this chapter, you will be able to:
- Explain how electrons are organized within an atom, and how this relates to chemical bonding and behavior
- Describe the different types of chemical bonds that hold molecules and compounds together
- Understand the different ways molecules are represented
- Understand the most common elements found in biological reactions
Chemical Bonds
Recall that the subatomic particles within an atom, the protons, neutrons, end electrons, change the nature and behavior of an atom. Every element has a fixed number of protons (for example, hydrogen has 1 proton and carbon has 6 protons), but the number of electrons and neutrons can very. For a quick refresher, take a look at this simulation on building atoms. The number of neutrons changes the isotope of an atom and can affect the stability of the atom. The number of negatively-charged electrons in an atom can change the charge of the atom. Here, we discuss how the number of electrons in an atom also controls the number and types of chemical bonds an atom can form.
Electron Shells
We can approximate the position of electrons inside an atom as being organized into different orbital shells surrounding the nucleus, where each orbital shell represents a different energy level. In reality, it is impossible to know the exact location of the electrons in an atom as they orbit the nucleus. As such, we make use of the concept of orbital shells that represent different energy levels as a useful approximation to understand how the number and state of electrons affect the behavior of atoms.
Figure 1 shows a graphical representation of electrons organized into orbital shells around neutral hydrogen (H), carbon (C), nitrogen (N), and oxygen (O) atoms, as represented by the chemical symbol for each element. Recall that for a neutral atom, the number of electrons matches the number of protons. Black, co-centric circles represent the orbital shells for each atom; one orbital shell is shown for hydrogen while two are shown for the other three elements. The smaller, innermost circle represents the lowest energy level. The outer circle represents a higher-energy level.
Only a certain number of electrons can exist at each energy level, which places a limit on the number of electrons that can occupy each shell. The innermost and lowest energy shell can contain only two electrons. The next energy level up can contain eight electrons.
The outermost orbital shell is known as the valence shell. Electrons in this shell are called valence electrons. It is in this valence shell where bonding between atoms most typically occurs via interactions between the atoms’ valence electrons. The chemistry of molecular bonds is driven by the stability of an atom with a filled valence electron shell. Atoms form chemical bonds so that they can completely fill their valence electron shell.
For example, in Figure 1 the pictured single carbon atom has four electrons in its outer valence shell. We know that this second orbital shell can hold up to eight electrons. A carbon atom with a valence shell completely filled with eight electrons is more stable, and therefore more chemical favored, than a valence shell with only four electrons.
Atoms will form chemical bonds to gain access to valence electrons that will fill their valence shells. When more than one atom bonds together, they form a molecule. These molecular bonds are typical divided into two categories: ionic bonds and covalent bonds.
Ionic Bonds
In an ionic bond, electrons are transferred from one atom to another. This results in two oppositely charged ions that are bonded by the attraction between opposite charges. For example, table salt is representative of a category of salts whose molecules are held together by ionic bonds. Table salt consists of bonded sodium (Na) and chlorine (Cl) atoms.
Sodium has one valence electron in its second orbital shell while chlorine has seven valence electrons in a valence shell that can hold eight electrons total. When sodium forms an ionic bond with chlorine, sodium “donates” its one valence electron to chlorine. Chlorine gains an electron, meaning it now has eight valence electrons and a fully filled valence shell. This transfer of electrons is shown in Figure 2.
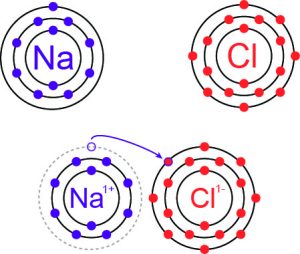
The sodium atom, whose valence shell is now the filled second orbital shell, has a slightly positive charge. With this extra electron from sodium, the chlorine atom now carries a negative charge. The attraction between the positively and negatively charged sodium and chlorine atoms (respectively) form an ionic bond.
Covalent Bonds
In a covalent bond, the electron orbitals of two or more atoms merge into a new system that allows electron pairs to be shared equally between more than one nucleus. Most chemical bonds in biological organisms are covalent bonds.
There are different types of covalent bonds depending on the number of shared electrons between the two bonded atoms. A single bond between two atoms shares a single pair of electrons, i.e. two electrons total are shared between the two atoms. A double bond shares two pairs (four electrons) and a triple bond shares three pairs (six electrons). Double bonds are shorter and harder to break than single bonds. Triple bonds even more so.
As with all chemical bonds, the details of how and when these bonds form is dependent on an atom’s valence electrons. Figure 3 portrays how two hydrogen (H) atoms and one oxygen (O) atom shares electrons to form a water molecule.
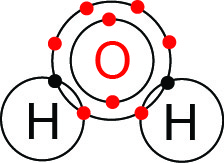
A neutral hydrogen atom has one proton and therefore one corresponding electron. With just one electron, hydrogen’s valence shell is the lowest energy orbital shell, which can hold up to two electrons. Hydrogen therefore has one valence electron and needs only one additional electron to complete its valence shell.
Oxygen, with eight electrons, has two electrons in the first electron shell and six valence electrons in the second, valence shell. Oxygen needs two additional electrons to fill its outer shell and can form two single or one double bond. In water, we see that oxygen shares electrons with two hydrogen atoms. From this, oxygen acquires access to the two additional electrons it needs to fill its valence shell. Each of the three atoms remains neutral, and each atom has a completely filled outer electron shell.
Chemical Formulas
The molecules, or chemicals, that are formed due to chemical bonding can be represented in a variety of ways. Figure 4 shows examples of different representations for methane, which consists of a carbon (C) atom bond to four hydrogen (H) atoms.
A molecular formula uses an element’s chemical symbol to indicate the types of atoms in a molecule followed by subscripts to show the number of atoms in the molecule. If there is no subscript, then there is only one atom of that element in the compound. With one carbon (C) atom and four hydrogen (H) atoms, methane’s molecular formula is CH4. Molecular formulas are used in chemistry to specify the molecule and to understand what atoms are needed to make up the molecule.
It is important to note that a subscript following a chemical symbol and a number in front of a symbol do not represent the same thing. For example, H2 and 2H represent distinctly different species. With a subscript, H2 is the molecular formula for two hydrogen molecules bonded to each other. On the other hand, 2H indicates two separate atoms of hydrogen that are not bonded together. The expression 2H2 indicates two molecules of diatomic hydrogen (see Figure 5).
The structural formula for a molecule shows how the atoms are connected in the molecule. Both a molecular and structural formula shows how many of each atom are in a molecule, but a structural formula more clearly indicates how the atoms are bonded together to form the molecule. For methane, the structural formula (see Figure 4 b) reveals that all four hydrogen atoms are bonded to a central carbon atom.
A ball-and-stick model shows the geometric arrangement of the atoms, which are not drawn to scale. As the name suggests, atoms are represented as balls and the bonds between atoms are represented as sticks. A ball-and-stick model expands on the bonds shown in a structural model by representing these bonds in 3D. For methane, the ball-and-stick model (see Figure 4.c) reveals that the four hydrogen atoms bonded to carbon form a pyramidal structure.
A space-filling model shows the 3D arrangement of atoms drawn to scale. It therefore is similar to ball-and-stick models, but incorporates information on atom size. For methane, the space filling model (see Figure 4.d) shows that the carbon atom is much larger than the hydrogen atom. We also see that the chemical bonds between the atoms do not protrude from each atom as suggested in the ball-and-stick model.
Empirical Formulas
Isomers
With more complex molecular formulas, a variety of isomers become possible. Isomers are compounds with the exact same molecular formula but different structures. Isomers are similar in spirit to homographs—words that have the same spelling but are pronounced differently (like minute, meaning 60 seconds, and minute, meaning small). Isomers cannot be differentiated by their molecular formula alone, it is necessary to use their structural formula to identify an isomer without ambiguity.
There are different types of isomers. Structural isomers describe isomers for which the atoms in the molecules are connected in completely different arrangements. These isomers tend to be exhibit completely different behavior. Stereoisomers describe isomers that are mirror-images of each other. It is important to consider the 3D geometry of molecules (for example with a ball-and-stick or space-filling model) to understand the differences in stereoisomers. Lastly, conformers are isomers that differ only in the conformation of the atoms in the molecule. In other words, though the atoms are bonded together in the same way, molecules can twist along single bonds, which result in molecules with different confirmations.
Building Molecules
Most atoms lack some valence electrons in their outermost electron orbital and thus have the potential to form a bond. In general, a molecule is “complete” or no longer prone to reacting when all there are no empty spots for electrons in any of the valence shells of the atoms in the molecule.
You can learn more about building molecules with this Molecule Building simulation!
CHNOPS: the Most Common Elements in Life
By mass, 95% of all biological systems on Earth are either carbon (C), hydrogen (H), oxygen (O), or nitrogen (N) atoms. This dominance is sometimes referred to as CHON. With phosphorous (P) and sulfur (S), which gives us CHNOPS, these six elements make up 98% of all life on Earth. By considering the valence electrons of these elements, we can understand how they behave and react in biochemistry.
Recall from our discussion of covalent bonds above that hydrogen has one valence electron and needs only an additional electron to complete its valence shell. Because hydrogen is so close to a full valence shell, it is highly reactive—it is easy to form a bond with hydrogen. With its one electron, hydrogen can form bonds wherever valence shells need to be completed; consequently, hydrogen makes up 59% of biological compounds.
Oxygen is short just two electrons of a full valence shell, which also renders oxygen atoms quite reactive. Oxygen is also very electronegative, meaning it can attract shared electrons towards itself. In covalently bonded molecules, this means electrons will spend more time near oxygen atoms than the atom on the other end of the bond. Oxygen in molecules therefore tend to carry a negative charge. In the example of water (see Figure 3), the oxygen atom is slightly negatively charged and the hydrogen atoms are slightly positively charged. This difference in charge makes oxygen a polar molecule.
Nitrogen, with seven electrons, has five valence electrons. This means nitrogen can form up to three bonds: three single bonds, or a single and a double bond, or a triple bond. All thee options are commonly found throughout organic chemistry. Oxygen’s ability to attract electrons and nitrogen’s relatively weak bonding of valence electrons drive many biochemical reactions. An example of the possible bonds formed by CHON is given in Figure 7, which diagrams the covalent bonds for a molecule called glycine.

Carbon is so central to organic processes that organic chemistry is sometimes defined as the study of compounds that contain carbon. With six total electrons, carbon has four valence electrons occupying its second electron shell. This allows carbon atoms to form four bonds or a combination of single, double, and triple bonds. Carbon-carbon bonds are particularly easily made and remain very stable. This allows carbon to form large and complicated structures, such as networked lattices (e.g., graphite or diamonds), cyclic structures, and other complex molecules used throughout biology.
Carbon’s ability to form four separate single bonds at once allows it to acquire the important property of chirality. Chirality is defined as the property of an object that can not be superimposed on its mirror image. The classic example, illustrated below in Figure 8, is your left and right hands. When both hands are palm up, they are mirror images of one another. If you lay your hands flat on a table, it is not possible to slide one hand over the other and match the other hand exactly. For this reason, chirality is often also referred to as “handedness”.

Carbon atoms exhibit chirality when they are simultaneously bound to four different things. In such a case, the carbon atom provides a chiral center. Chirality can have a huge impact on the reactivity of a molecule in biological systems. Further details and an example is give in the next chapter, which discusses chirality in amino acids (link to anchor/section).
Phosphorus and sulfur, while relatively less abundant, are nonetheless essential to life as we know it. Phosphorus is involved in forming the borders between cells, the back bone of genetic material, and energy storage and transport in cells. Sulfur helps to ensure proteins maintain their shape and carry on important functions. These key biochemicals are discussed more in the following chapter.
Key Concepts and Summary
Biochemistry is driven by electrostatic forces to share electrons of elements as a way of balancing positive and negative charges. The electrons in covalent bonds are shared equally between two or more atoms, while ionic bonds essentially transfer a loosely bound electron to fill the valence shell of another atom. The most common elements used by life on Earth are carbon, hydrogen, nitrogen and oxygen as well as phosphorus and sulfur (CHNOPS). Carbon is central to organic processes with half of its outer valence shell empty and carbon easily forms single, double and triple bonds with other elements. Just two electrons short of filling its outer valence shell, oxygen is highly reactive, driving many reactions in geology and biochemistry.
Review Questions
Exercises
- Use Molview, a free-to-use software that will allow you to build molecules (especially organic molecules) to learn about the structures of simple and complex molecules. Choose a molecule and investigate how modifying different atoms included in it will affect the structure of the molecule. Find molecules in this text or in other resources that are important for life and see if these molecules are included in its database. Alternatively, build your own molecule using its chemical formula.
Summary Questions
- How does the electronic structure of atoms affect the way atoms bond?
- What is the difference between ionic and covalent bonds?
- What information is contained in a chemical formula? What do the different letters and numbers in a chemical formula indicate?
- What are CHNOPS? How are they important for life?
- What is chirality and how does it affect the way different compounds are used by living things?