15 Earth Characteristics
The Earth is filled with a rich diversity of life. What makes the Earth such a habitable oasis for life? The planets Venus, Earth, and Mars all started out with atmospheres, and yet they evolved into vastly different worlds today. Certain features unique to the Earth, such as plate tectonics, have enabled it to be a dynamic world with oceans and a climate hospitable for life. By identifying the characteristics that make Earth hospitable, we can gain insight into what properties to look for on exoplanets that may point to the possibility of life.
Learning Objectives
By the end of this chapter, you will be able to:
- Discuss the Earth’s density and surface gravity and how it compares to the other planets in our solar system
- Explain the differentiation of Earth and its main interior layers
- Discuss how heat is generated inside a planet and how it is lost to cooling.
- Discuss the importance of plate tectonics for Earth’s habitability
- Explain how Earth’s magnetosphere protects life on Earth
The size of a world and distance it orbits its host star can take us very far in assessing whether the world could host life. We say more in the chapter on Habitable Zones about how the distance of the Earth from the Sun keeps the Earth at the right temperature for liquid water — and makes Venus too hot today for liquid water — and we will focus here on the bulk properties of the Earth.
Bulk Properties of the Earth
The most basic measurements of the Earth are its size, mass, and density. Many features of the Earth have been understood since antiquity. Aristotle argued that the Earth had to be round because the Earth cast a circular shadow over the moon during a lunar eclipse. Eratosthenes of Cyrene obtained a clever measurement of the radius of the Earth that was within 20% of the value that we can measure today. To a good approximation, the Earth is spherical in shape and the volume of the spherical Earth is given by $\frac{4}{3} \pi R^3$, where $R$ is the radius of the Earth.
Armed with knowledge about the volume of Earth, we can use the density of rocks to estimate the mass of Earth. Density is simply the ratio of mass to volume, $\rho = M/V$, and it tells us how tightly material is packed into an object of a given size. A rock that is about a cubic centimeter in size weighs about 3 grams, so the density of that rock is $\rho$ = 3 g/cm3. We can then turn the density equation around to estimate the mass of the Earth: M = $\rho$V. This estimate is off by a factor of about two since the interior of the Earth is compressed and has a higher density than surface rocks. However, this “order of magnitude” estimate is an excellent approximation – a factor of two is a great start when nothing is known before.
In the early 18th century (and with the benefit of insights from Isaac Newton about the laws of gravity) Henry Cavendish “weighed the Earth” in his lab by cleverly measuring the acceleration of gravity on Earth. Gravity is a property of mass and Cavendish calculated a bulk density for the Earth of 5.48 g/cm3, very close to the currently known value of 5.515 g/cm3.
The density of a planet can easily be calculated if the radius and mass are known, quantities that can be measured even for planets orbiting other stars. The density of a planet gives immense insight into that world. All terrestrial planets in our solar system have higher densities than the jovian gas giants. The average density of the terrestrial planets is 5.0 g/cm3, while the average density for the jovian gas giants is 1.2 g/cm3. Exoplanets with densities that fall in between these values are commonly found and point toward intermediate types of planets that are not found in our solar system. For example, the “mini-Neptune” K2-18 b has an estimated density of 2.6 g/cm3.
Worked Example: Calculating the density of a planet
Looking up the mass and radius of the Earth, and using the density equation we find:
$\rho = M/V = M/\frac{4}{3} \pi R^3$ = (5.97×1024 kg)/($\frac{4}{3} \pi$ (6.38×106 m)3) = 5500 kg/m3
This tells us that an average cubic meter of the Earth contains 5500 kg, or 5.5 metric tons of matter. A cubic meter is about the size of a washing machine. If 5.5 tons seems heavier than you would have guessed, it is because the core density of Earth is greater than the dirt and rocks on the surface of our planet.
It can be more convenient to express densities in units of g/cm3 (grams per cubic centimeter). In these units the density of water is 1 g/cm3 and makes an easy reference point to remember. In these units, $\rho_{Earth}$ = 5.5 g/cm3
Extra: Saturn has the lowest density in our solar system, with a value of $\rho_Saturn$ = 0.7 g/cm3. How many times denser is the Earth than Saturn?
Show Answer
7.9 — the Earth is almost 8 times more dense than Saturn
Another property that also depends on the mass and radius of a planet is surface gravity. The equation for finding the surface gravity (g) of a world is: $g=G M/R^2$. Here, $G$ is the gravitational constant and we can easily make comparisons of the surface gravities of different worlds by taking their ratio relative to Earth. Table 1 lists the densities for the planets in our solar system and their surface gravities relative to the surface gravity of Earth. The surface gravity helps to assess if rocky planets can hold onto their atmosphere.
Mercury | Venus | Earth | Mars | Jupiter | Saturn | Uranus | Neptune | |
ρ (g/cm3) | 5.4 | 5.2 | 5.5 | 3.9 | 1.3 | 0.7 | 1.3 | 1.6 |
g (relative to Earth) | 0.38 | 0.91 | 1 | 0.38 | 2.4 | 0.92 | 0.89 | 1.1 |
The size of a world is also directly related to how fast it cools down. All planets start off in a molten state and over time the energy is radiated away into space. The cooling time of an object depends on the ratio of the surface area to the volume ($\frac{SA}{V}$) of a planet. Since the surface area of a sphere is $SA = 4\pi R^2$ and the volume of a sphere is $V = \frac{4}{3} \pi R^3$, this means that the cooling time is proportional to $\frac{1}{R}$. This means that there is an inverse relationship between the cooling time for a world and its radius, and a smaller world cools off faster than a world with a larger radius. How quickly a world loses its heat (putting aside for a moment the question of what creates the heat inside of a world) can be understood with a simple example from everyday life. Consider a large, hot baked potato and a small piece cut off the larger potato: which cools first? Intuition tells us it is the smaller piece, and this intuition is correct. In our solar system, the worlds with the smallest size indeed have cooled the fastest: the asteroid Ceres, the Moon, and Mercury have all entirely lost their internal heat and are geologically dead.
The fact that smaller worlds lose their internal heat (or cool off) more quickly than larger worlds is a fundamental reason why the Earth is hospitable to life. The Earth still has heat inside that can power geological activities such as plate tectonics that stabilize our atmosphere.
Earth’s Interior
How do we know about the interior structure of Earth, since we live on the surface? We can only drill a few kilometers before the temperature and pressure become too high to continue. The deepest manmade hole on Earth is the aptly named the Kola Superdeep Borehole, which reaches down to 12.3 km (7.6 miles).
Almost everything else we know about the interior of the Earth comes from seismic waves. Seismic waves carry the energy from events like earthquakes or volcanic eruptions. Seismic waves compress the elastic interior of the Earth like sound waves compress the air. The speed of seismic waves depends on the depth and density of medium. The fastest moving P-waves compress material in the direction that they travel. They are the first to arrive at seismic stations and travel through the core of the Earth. Sheering S-waves are slower and do not propagate through liquid material; they are blocked by the liquid core of the Earth. Slower still, R-waves ripple along the surface of the Earth. They arrive last at the seismic stations but have the potential to do the most damage.
Seismic waves reveal the bulk density structure of the Earth, as depicted in Figure 2 below. The core is about half the radius of the Earth and is comprised of an inner core that is about the size of the moon, surrounded by a molten iron core. The liquid core is also an important factor in the Earth’s habitability, as it enables the Earth to have a protective magnetosphere surrounding it. The thick rocky mantle wraps around the core and extends for the other half of the Earth radius. The outer shell is a thin crust of low density rock called the lithosphere.
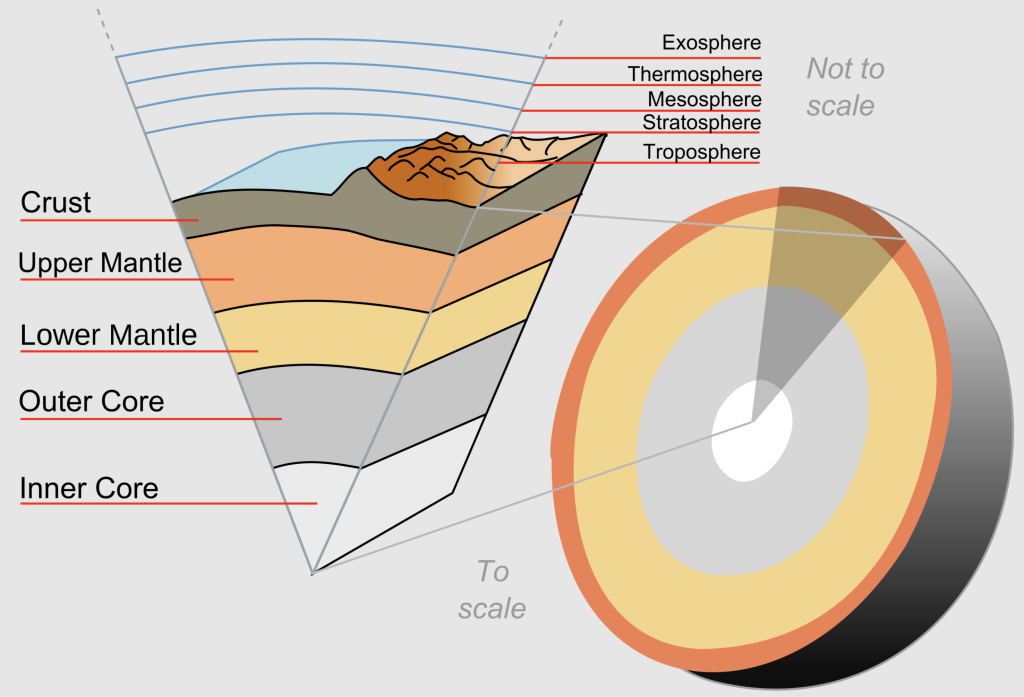
All terrestrial planets in our solar system appear to have a similar layered structure inside, with a dense metal core at the center surrounded by the mantle and crust. This layered structure occurs because the planet cools over time and as it cools, the process of differentiation occurs, where the densest materials (like iron) sink to the center and the lightest materials float to the surface. A simple real world example shows this to be an intuitive process. If you shake oil and water together in a container, they will initially be in a mixture. However, over time, the denser water sinks to the bottom of the container and the lighter oil rises to the top.
One feature that distinguishes Earth from the other terrestrial planets is its crust. The Earth’s crust comprises two types: continental crust and oceanic crust. These are the tectonics plates that literally float and move around on top of the mantle, specifically on top of the asthenosphere. Earth is the only terrestrial planet that has oceanic crust so this gives us a clue that plate tectonics may be an important consideration when assessing the habitability of an exoplanet.
Sources of Planetary Heat
The Earth is a geologically active planet. Plate tectonics has already been mentioned as one of the unique properties that the Earth has compared to the other terrestrial planets in our solar system and a source of heat is required to drive this geological activity. There are a few possible origins for heat inside of a planet: accretion energy, differentiation and radioactive decay.
Planets form by accreting planetesimals that collide and stick together to form larger bodies. These collisions generate significant amounts of heat or accretion energy as the planet is assembled. However, all of the heat from accretion energy is injected during the early stages of planet formation.
As material sinks during differentiation, energy is conserved as gravitational potential energy is converted first to energy of motion (kinetic energy) and then to heat (thermal energy).
Over time, as differentiation is complete and accretion is much less frequent, radioactivity becomes and remains the main source of interior heat for a planet (we note here that the large jovian planets still have heat from their formation in their voluminous interiors). Radioactivity is a natural process in which heavy nuclei spontaneously break apart; when the nuclei break apart into smaller ones, energy is emitted. All planets in our solar system contained the same chemical mixture from the solar nebula at early times, and these include some heavy radioactive elements such as uranium (238U) and potassium (40K).
Radioactivity continues to supply internal heat to the Earth to fuel geological activity, yet Mercury and the Moon are geologically dead. The difference here goes back to the cooling rate of a planet. Smaller planets lose their heat faster than larger ones. Therefore, the size of a world is an important indicator of the potential for geological activity. Before describing types of geological activity, let’s consider exactly how heat escapes from inside of a planet.
Heat Loss From a Planet
The interior heat of a world can be lost via several mechanisms: convection, conduction, and radiation. When heat from the core moves to the lower mantle boundary, convection cells are set up inside of the mantle. Convection is the familiar process wherein hot material, such as air, rises as cooler material sinks. Convection, in which energy is transported from a warm region, such as the interior of Earth, to a cooler region, such as the upper mantle, is a process we encounter often in astronomy—in stars as well as planets. You can see convection in action when boiling a pot of water on a stove. As the water heats up from below and starts to boil, convection cells are set up in the water, as seen in Figure 3. Once the heat reaches the top of the mantle, it then moves through the crust via conduction. Finally, the heat makes it to the Earth’s surface and is radiated away into space (or, to be specific, in the case of planets with atmospheres, like the Earth and Venus, the heat must travel through this layer first).

Now that the mechanisms for generating heat inside a planet, as well as how that heat gets out of a planet, are understood, we can move on to discuss the different types of geological activity that can be driven by this internal heat.
Plate Tectonics
The size of the Earth, specifically its radius, is fundamentally the reason why the Earth is still geologically active: there is enough internal heat inside our relatively large planet to drive geological activity. A smaller terrestrial planet, such as Mercury, is too small to retain enough heat to sustain any geological activity: Mercury cooled off fast and is now geologically inactive.
An important property of Earth is that it has plate tectonics. Plate tectonics explains how slow motions within the mantle of Earth move large segments of the crust, resulting in a gradual “drifting” of the continents as well as the formation of mountains and other large-scale geological features. The power to move the plates is provided by slow convection of the mantle, a process by which heat escapes from the interior through the upward flow of warmer material and the slow sinking of cooler material.
While Venus and Mars are likely to also have convection in their mantles, Earth is the only planet in our solar system known to have plate tectonics. Geological evidence suggests that plate tectonics began operating on the Earth about 3.8 billion years ago. The upper rocky layer of the Earth is divided into about twelve major tectonic plates that float on top of the convecting mantle, shown in Figure 4. The distribution of these plates affect global climate because continental crust has a higher albedo (reflects more light) than ocean water. Continental crust was thought to have a maximum extent between 1.6 – 2.7 billion years ago, causing ice ages and high rates for burial of organic material in the early and late Proterozoic eon.
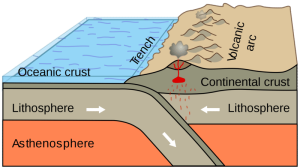
Plate tectonics provide a mechanism for circulating material between the surface of the planet and Earth’s interior. Plates pull apart from each other along rift zones, such as the Mid-Atlantic ridge, driven by upwelling currents in the mantle. Subduction zones form at boundaries where one edge of an approaching plate moves under another. These recycling zones are critical for life on our planet. They are part of a negative feedback loop that stabilizes our climate thanks to chemical interactions between surface rocks and the atmosphere. Rain removes carbon dioxide from the atmosphere and stores it as calcium carbonate in surface rocks through a weathering process. Carbon-enriched rocks are then transported into the deep mantle along the subduction zones, removing excess CO2 from the atmosphere. The flow of material goes the other way as well; some elements are transported from the mantle to the surface of the planet.

Volcanoes are also formed through plate tectonics. When volcanoes erupt, gasses trapped inside of the Earth are released. This process of volcanic outgassing supplies the raw materials for the Earth’s atmosphere (Figure 6). The primary materials that are outgassed include water vapor, CO2, N2, and SO2. Some of these gasses are greenhouse gases and contribute to maintaining the energy balance of the Earth. Plate tectonics on Earth allows carbon to be cycled from the atmosphere, down to carbonate rocks on the ocean floor, and back into the atmosphere through volcanism. This carbon cycle is an essential part of maintaining a hospitable atmosphere on Earth. Other planets in our solar system experience tectonic stresses, but plate tectonics – with the active movement of crustal and oceanic plates – is unique to Earth. When exploring exoplanets that may be conducive to life, the presence of plate tectonics would be a favorable condition, at least based on our experience on Earth.
Earth’s Magnetosphere
The core of the Earth has a temperature similar to the surface of the Sun. This heat ionizes the molten iron, producing charged particles. As the liquid metal inside Earth circulates, the charged particles set up an electric current. When many charged particles are moving together like that—in the laboratory or on the scale of an entire planet—they produce a magnetic field. Earth’s magnetic field extends thousands of miles above the surface of the planet.
Our planet behaves in some ways as if a giant bar magnet were inside it, aligned approximately with the rotational poles of Earth. When charged particles from the surface of the Sun (the “solar wind”) are directed toward us, the Earth’s magnetic field deflects them and shields the planet. This region, called the magnetosphere, is defined as the zone within which Earth’s magnetic field dominates over the weak interplanetary magnetic field that extends outward from the Sun (Figure 7). The magnetosphere plays an important role for habitability, by preventing stripping of our atmosphere.
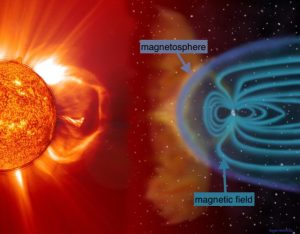
For a planet to have a magnetic field, it must have a liquid metallic layer and it must also be spinning fast enough to create a turbulent flow. Jupiter meets both of those conditions — it has a liquid conducting layer made of liquid metallic hydrogen in its outer core and it rotates once every 11 hours — and has the strongest magnetic fields in our solar system. Although Venus has a liquid metallic outer core like the Earth, it rotates too slowly on its axis (once ever 243 days) to generate a magnetic field. When assessing the habitability of an exoplanet, we want to determine whether it has the conditions to set up a protective magnetosphere.
There is evidence in Earth’s geologic record from magnetic minerals that many magnetic pole reversals have occurred in the past and some scientists believe that the magnetic fields are beginning to collapse and flip again. Life has existed on our planet for billions of years, and no correlation has been found between mass extinctions and magnetic pole reversals. The risk to life during a magnetic pole reversal may be modest, but the risk to power grids that modern humans depend upon will be more substantial.
Earth’s Habitability
We have looked at some of the properties of Earth that contribute to making it a habitable world. We began this chapter by stating that the Earth’s size and distance together can explain some of the unique properties that make Earth habitable. The Earth has a large enough size to have heat inside of it, initially from accretion and differentiation, but mainly from radioactivity today. As this heat escapes from the Earth, it is carried from the lower to the upper mantle through convection. This heat at the upper mantle powers plate tectonics, which is responsible for maintaining a continuous cycle between the Earth’s atmosphere and the crust, thus setting up a stable climate. One of the Earth’s differentiated layers is the liquid outer core, and this layer combined with Earth’s rotation rate sets up a protective magnetosphere around our planet.
Key Concepts and Summary
The Earth formed through the collision of planetesimals in our solar system when the Sun was forming more than 4.5 Gya. The planetesimal collisions deposited frictional accretion energy in the interior of our planet and heavier elements in the molten young Earth began to settle toward the core, differentiating into layers. The frictional processes of differentiation delivered an additional boost of energy to the interior. Today, Earth has a solid iron core surrounded by a molten liquid outer metal core. Turbulence in the outer core is coupled with rotation of the planet to generate a global magnetic field that shields the atmosphere from being eroded by the fast-moving particles in the solar wind. The energy of accretion and differentiation were one-time processes that deposited internal heat to the planet long ago. Now, an additional source of energy comes from the radioactive decay of heavy elements. The internal heat beneath the lithosphere drives motion of the floating continental plates. Planets lose internal heat over time (with smaller planets cooling faster).
Review Questions
Summary Questions
- What two properties of a world determine its density?
- How does the density of the Earth compare with the other planets in our solar system?
- How does surface gravity differ from density?
- Can a planet with a low surface gravity hold on to an atmosphere?
- Where did heat inside the Earth come from initially? What is the main source of heat from inside the Earth today?
- How can heat escape from inside a planet to the surface?
- What property of a planet determines how quickly it will lose its interior heat?
- Which planet cools faster: Earth or Mars? Explain.
- How does plate tectonics contribute to Earth’s habitability?
- Can a planet without plate tectonics be habitable?
- How does Earth’s magnetosphere protect life?