12 Development of Life on Earth
Billions of years ago the Earth was a very different place. Life seems to have emerged almost as soon as conditions were favorable after the planet had cooled and the environment allowed for the prebiotic chemistry to happen. As life proliferated, it had a profound effect on our planet affecting the environment, climate, and composition of our atmosphere while leaving behind a fossil record of its presence. In this chapter, we will explore the development of life and what evidence we have for how these changes occurred across geologic time.
Learning Objectives
By the end of this chapter, you will be able to:
- Discuss the evidence for where and when the first life on Earth may have arisen
- Describe hydrothermal vent communities
- Explain how oxygen became a key component of the Earth’s atmosphere and life processes.
- Describe the different geological timeframes and how they relate to the development of life on Earth
Where did life arise on Earth?
It is unclear whether the transition from chemistry to biology occurred on the land surface of Earth or in the oceans. Those intermediate species on our planets – entities that are more than chemistry, but less than life – no longer seem to exist.
In the mid-1960s, Alexander Graham Cairns-Smith proposed that complex prebiotic molecules may have first organized around the regular crystal patterns found in minerals in clay. Cairns-Smith hypothesized that electrostatic forces in mineral crystals would help to concentrate and align specific molecules to their surface. With the help of this clay, precursors of RNA could have assembled, triggering the start of an RNA world. Tide pools have also been proposed as the site where chemistry developed the complexity needed for life. The cycles of evaporation and subsequent addition of water in tide pools might have concentrated the organic materials necessary to form life. Higher concentrations increase the likelihood that these organic materials will react and form RNA or lipids.
It is possible that, far from the rocky surface of Earth, life originated deep in the oceans when our planet was a frozen world. During the phases where Earth was covered by a thick mantle of ice, the ice may have protected organic compounds beneath the ice from the damage of impacting asteroids or ultraviolet radiation from the sun.
Deep-sea hydrothermal vents are hot spots that release gas from the Earth’s interior. DNA sequencing suggests that LUCA was a thermophilic microbe and deep sea vents might have been ideal locations for nurturing that early life. At first glance, this seems an inhospitable place for life. These vents are located in some of the darkest, highest pressure environments on our world. However, the gas that is released from hydrothermal vents creates a natural chemical gradient that can be used by living cells to generate the energy needed for metabolism. In deep hydrothermal vents, this chemical gradient flows around the rocks surrounding the vent and creates nooks where organic compounds can collect and reach higher concentrations. Minerals that are capable of acting as catalysts have been found in deep sea vents. Therefore, hydrothermal vents seem to provide the needed ingredients: high concentrations of organic compounds, natural catalysts, and a powerful energy source in the absence of sunlight. Today, extremely varied ecosystems are found around these vents.
When did life arise on Earth?
The evidence for timing the initial rise of life on our planet is ambiguous. The most ancient evidence has been destroyed as the first forms of life would have been single-celled organisms that did not leave behind fossil remains. However, suggestive evidence from biology, chemistry, and geology supports estimates that life arose on Earth between 3 to 4 billion years ago. Since the Earth formed about 4.56 billion years ago, the early appearance of life hints that the evolution from chemistry to life may be statistically probable. If so, this greatly increases the odds that life has also evolved elsewhere.
Carbon isotopes
The earliest line of evidence for timing the rise of life comes from carbon isotopes. Carbon naturally occurs in three isotopes, always with 6 protons, but with either 6, 7, or 8 neutrons, which are annotated 12C, 13C, and 14C respectively. The superscripts indicate the atomic mass number, or the total number of protons and neutrons in an element. Of these isotopes, 14C will undergo radioactive decay with a half life of 5730 years. However, 12C and 13C do not decay and therefore, the ratio of 12C to 13C is constant over time on the Earth. However, life preferentially incorporates the lighter 12C, rejecting 13C. Therefore, a low ratio of 13C to 12C offers a tentative suggestion that organic material existed and that isotopic imbalance can be incorporated into the metamorphic structure of the rock.
Why does life prefer to use 12C? While the chemical reactivity of an atom is predominantly driven by the electron configuration, more massive isotopes tend to have slightly slower reaction rates. That slight edge in the speed of reaction rates is enough of an advantage to favor the uptake of carbon-12 over carbon-13 in organic biochemistry.
Zircon crystals are commonly used to assess ages in very ancient geological records because they are very durable minerals, resistant to both heat and corrosion. Trapped minerals can be preserved when zircons form. A group of scientists from the University of California, Los Angeles studied 10,000 zircons gathered from western Australia. Of these ten thousand zircons, one contained a graphite inclusion, a compound composed entirely of carbon atoms.
Radiometric dating showed that this zircon crystal was 4.1 Gyr old and the carbon inclusion exhibited a larger ratio of 12C to 13C. Was this the chemical stain of primordial life, dating back to 4.1 Gya? On Earth today, this would be a good indicator of organic material. But, interpreting this result from so long ago is more controversial.
Stromatolites
Ancient stromatolites offer a more secure timeline for the emergence of life. These fossilized structures are found in shallow waters and look like modern structures formed by cyanobacteria. The oldest stromatolites have been found in South Africa and Australia and date back to the early Archean Period between 3.2 – 3.5 Gya. By the end of the Archean and throughout the Proterozoic geological periods, stromatolites appear to have been abundant and formed the first reefs.

Stromatolites today are composed of layers of microbial mats of photosynthesizing cyanobacteria, as shown in the YouTube video below. Photosynthesis depletes carbon dioxide in the water, precipitating calcium carbonate deposits, which along with other sandy sediments, is trapped in the sticky bacterial film. The bacterial colonies grow upward towards better exposure to the sun, and over time the layers of bacterial film and mineral precipitates build up to form distinctive layered stromatolites.Similar stromatolite structures are formed today.
We guess that ancient stromatolites were formed through biological processes, since we observe this behavior in microbes today. The processes required to make these structures seem to require microbes that are already quite complex and capable of photosynthesis. Actual first life then, likely to be a more primitive organism, would have existed even earlier than these ~3.5 Gyr old structures.
Microfossils
The most direct evidence for life comes in the form of microfossils, fossils preserving micro-organisms that may have been among the first living creatures on Earth. The most convincing microfossils date back to approximately 3 billion years ago. However, these are difficult to identify both because rocks undergo erosion over time and because the structures that resemble microfossils might actually be formed by nonbiological processes. Many supposed microfossils turn out to be false positives after careful chemical analysis. Though the discovery of a microfossil is an unmistakable mark of life, it is easy to be fooled. The Figure below gives you a good idea of the scant information content in microfossils.
The Age of Life
Evolution and Extinction
On geological time scales, time is organized into eons, eras, and periods. There are four main eons subdivided into different eras. The eras are in turn split into different periods. The current eon, the Phanerozoic Eon (541 Mya through today), is known as “The Age of Life.” The Cambrian Period is the earliest period of the Phanerozoic eon, lasting from 540 to 500 Mya. The events leading up to this period set the stage for an explosion of complex life. Changing atmospheric oxygen concentration allowed for more efficient aerobic metabolism and increasingly complex life forms.
The transitions between different geologic periods are defined by changes in the fossil record with many of the most dramatic changes occurring during mass extinction events, defined as a period of time when the rate of species going extinct is increasing with respect to the rate at which new species arise. Throughout the Phanerozoic era, there were 15 documented major extinction events. Five of these were major mass extinction events where more than half of all species on Earth at the time were lost. While mass extinctions are devastating, they do serve to encourage diversity. Had the dinosaurs not been eliminated 65 Mya, there may never have been such a successful emergence of mammals. The frequency of life in the Universe is a function of how often it arises, how often it survives, and how long it endures after evolving. In this way, the frequency and intensity of mass extinctions control the frequency of life.
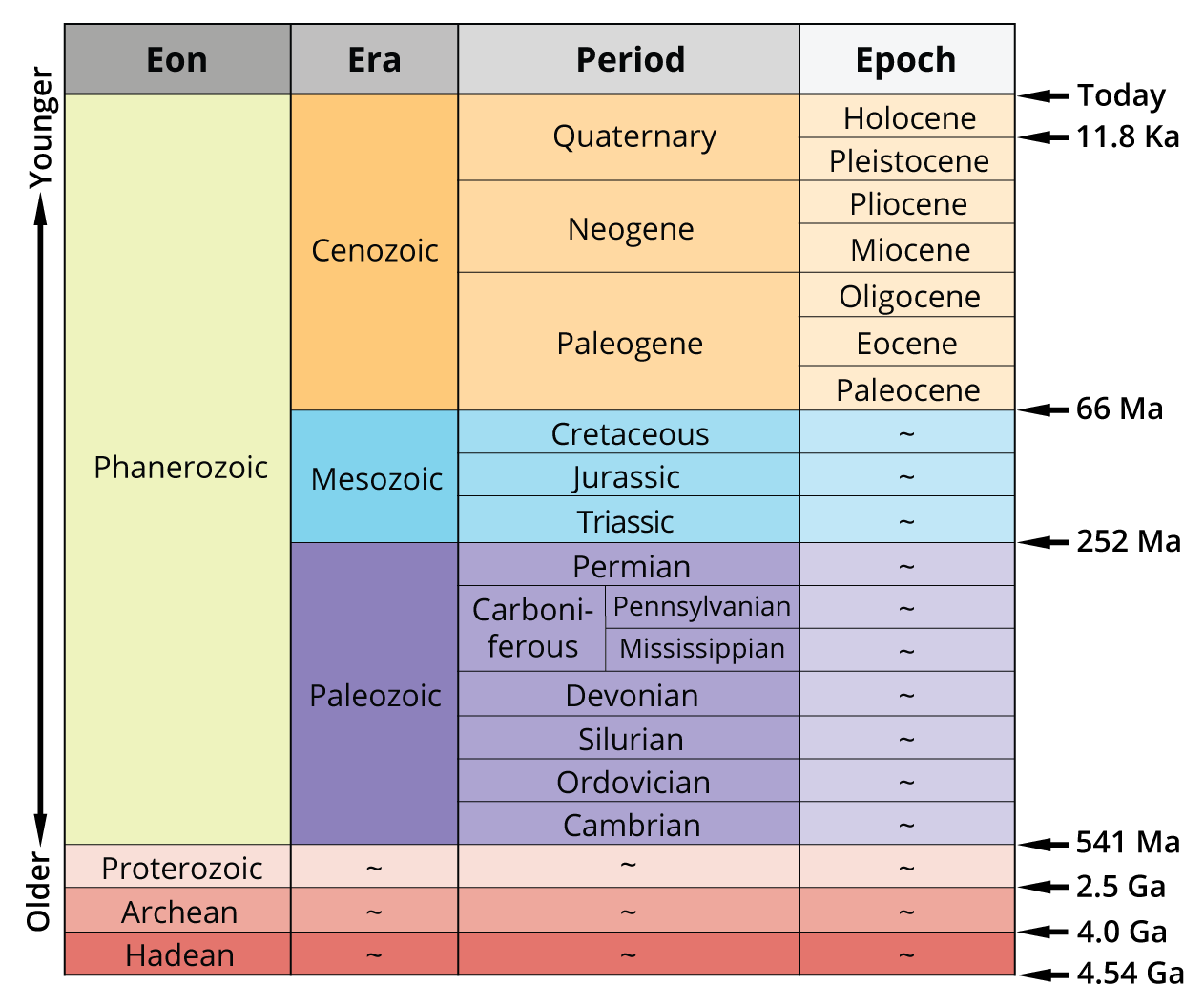
Precambrian Life
The Proterozoic Eon began 2.5 billion years ago and set the stage for Phanerozoic life. From the geologic record, we know that oxygen levels were low before the Proterozoic. Therefore, microbes from the start of this era would have primarily been anaerobic chemotrophs, producing energy through chemical pathways.
Want to know more: Metabolic Lifestyles
Organisms can be identified according to the source of carbon they use for metabolism as well as their energy source. The prefixes auto- (“self”) and hetero- (“other”) refer to the origins of the carbon sources various organisms can use. Organisms that convert inorganic carbon dioxide (CO2) into organic carbon compounds are autotrophs. Plants and cyanobacteria are well-known examples of autotrophs. Conversely, heterotrophs rely on more complex organic carbon compounds as nutrients; these are provided to them initially by autotrophs. Many organisms, ranging from humans to many prokaryotes, including the well-studied Escherichia coli, are heterotrophic. All pathogens are heterotrophic because their carbon source is their host.
Organisms can also be identified by the energy source they use. All energy is derived from the transfer of electrons, but the source of electrons differs between various types of organisms. The prefixes photo- (“light”) and chemo- (“chemical”) refer to the energy sources that various organisms use. Those that get their energy for electron transfer from light are phototrophs, whereas chemotrophs obtain energy for electron transfer by breaking chemical bonds. There are two types of chemotrophs: organotrophs and lithotrophs. Organotrophs, including humans, fungi, and many prokaryotes, are chemotrophs that obtain energy from organic compounds. Lithotrophs (“litho” means “rock”) are chemotrophs that get energy from inorganic compounds, including hydrogen sulfide (H2S) and reduced iron. Lithotrophy is unique to the microbial world.
The strategies used to obtain both carbon and energy can be combined for the classification of organisms according to nutritional type. Most organisms are chemoheterotrophs because they use organic molecules as both their electron and carbon sources. Table 1 below summarizes this and the other classifications.
Classifications | Energy Source | Carbon Source | Examples | |
---|---|---|---|---|
Chemotrophs | Chemoautotrophs | Chemical | Inorganic | Hydrogen-, sulfur-, iron-, nitrogen-, and carbon monoxide-oxidizing bacteria |
Chemoheterotrophs | Chemical | Organic compounds | All animals, most fungi, protozoa, and bacteria | |
Phototrophs | Photoautotrophs | Light | Inorganic | All plants, algae, cyanobacteria, and green and purple sulfur bacteria |
Photoheterotrophs | Light | Organic compounds | Green and purple nonsulfur bacteria, heliobacteria |
Source: LibreTexts Biology
The Proterozoic Eon was likely a time of rapid diversification of life. Today, there are systems of proteins that run checks during the replication of DNA to minimize transcription errors. However, these types of proteins are less common in prokaryotic microbes, which were common during the Proterozoic Era. A higher rate of mutations would have accelerated natural selection and driven a greater diversification of organisms. One of those mutations enabled photosynthesis, a more efficient process of respiration, resulting in an explosion of complex multi-cellular organisms that appeared at the start of the geologic era known as the Cambrian Explosion.
The Cambrian Explosion
At the time of the Cambrian explosion, there was an exponential increase in the number and complexity of organisms. Complex organisms likely preferred living in the oceans at first, where there was easy mobility and easy access to nutrients. Even very shallow levels of water provide protection from damaging UV radiation.
The earliest evidence of complex, land-based life comes from early land plants that begin to appear in the fossil record around 475 Mya. DNA evidence suggests that these plants evolved from algae, a general term for aquatic, photosynthetic organisms. It is a likely transition given that many breeds of algae prefer growing in shallow water to begin with. Algae adapted protections, such as thicker cell wells in order to survive dry spells, that would have been beneficial in the transition from water to land.

With other organisms still constrained to the water, land plants flourished and grew large in size. The lack of competition further allowed for increasing complexity: the development of root systems and tubes to transport water and nutrients throughout the plant. Photosynthesizing land-based plants had abundant energy from the Sun.
The Carboniferous Period
Forests began to cover the lands and dead organic matter began to pile up and decompose among the plants. Millions of years later, this organic matter would be compressed into coal, earning this period the name “carboniferous,” which means “coal carrying” in Latin.
The Carboniferous period began roughly 360 Mya and lasted about 60 million years. It was marked by an era of giantism. The fossil record from early in this period is rich in ocean and fresh water organisms. Land-based organisms appeared in the middle to later part of the period. The first terrestrial organisms were supersized insects and amphibians. By the middle of the Carboniferous period, adult amphibians were up to 6 meters long and developed the scaly skin typical of lizards by the end of the period. The Arthropleura, which resembles a 2.6-meter long millipede, proliferated during the late Carboniferous period and remains the largest-known land invertebrate ever discovered.
The Carboniferous period also gave rise to the largest-known flying insect: the Bolsover dragonfly. The Bolsover dragonfly looks nearly identical to the dragonflies common today, but with a wingspan of 30 cm – six times larger than a modern dragonfly. With a wingspan this large, the Bolsover dragonfly would not be able to fly in today’s atmosphere, with 21% oxygen. This has been used to suggest that atmospheric oxygen levels may have been as high as 30% during the Carboniferous period. Increased levels of oxygen as well as a generally moister environment would explain the large size of organisms at the time. Respiration was likely more efficient so that organisms could produce more energy and sustain their larger sizes.
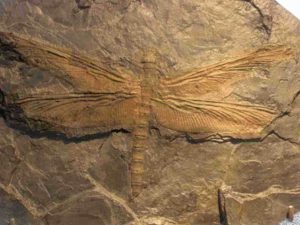
As life continued to evolve and diversify, ecosystems began to resemble the types of settings we are familiar with today. This penchant for giantism continued into the Mesozoic era, which spanned from 252 to 66 Mya and is known as “the Age of the Dinosaurs.” The Cenozoic era that followed saw the emergence of mammals. The end of each period during the Phanerozoic Eon was marked by mass extinction events that completely upended the biological landscape of the time.
Great Oxidation Event
The Great Oxidation Event is alternatively called the Oxygen Catastrophe depending on whether you ask aerobes or anaerobes. It marks a drastic change in the Earth’s atmospheric composition that occurred about 2.5 Gya.
An increase in atmospheric oxygen is seen in the geological record as a sudden onset of oxidation of iron occurred. Iron oxidation coincides with the geological evidence for glaciation 2.5 billion years ago. Oxygen is not a greenhouse gas, but it is a highly reactive species that would have interacted with the primitive methane atmosphere produced by volcanic outgassing. Methane is a powerful greenhouse gas. As oxygen levels rise, oxygen would react with methane to form carbon dioxide and water. This mechanism for removing methane would have produced the significant cooling and widespread glaciation. An outcrop of ancient Canadian rocks shows evidence of a glaciation event between different rock layers. Below (and therefore older than) this glaciation event, the rocks are consistent with low levels of atmospheric oxygen. Younger rock above the glaciation layer show significantly higher oxidation. Radiometric dating of the rock show that the increase in atmospheric oxygen, or the Great Oxidation Event, occurred 2.45 billion years ago.
The addition of oxygen to the atmosphere is believed to be the result of photosynthesis from cyanobacteria. Oxygen did not accumulate immediately because of the enormous number of sinks, including reduced gases and minerals that would have overwhelmed its production. Those sinks for oxygen are now largely saturated; respiration of anaerobic organisms (including us) and decay of organic material take up most of the oxygen produced today.
As the atmosphere cooled, a positive feedback loop ensued. Water vapor, which is another important greenhouse gas, would have condensed out of the atmosphere. The Earth eventually froze over and became what is known as ”snowball Earth.” Volcanic activity would have continued during this phase, releasing internal heat along with carbon dioxide into the air. With a sufficient amount of CO2, the greenhouse warming would have melted the ice, allowing the Earth to recover from its frozen state. Assuming present rates of volcanism, the necessary build up of CO2 would take 10 million years. Evidence in the rock record suggests that one snowball Earth event occurred around 2.2 billion years ago and another series of snowball Earths that happened just prior to the Cambrian Explosion.

Key Concepts and Summary
The deep oceans may have been one of the most stable environments on Earth, immune to the wild swings of conditions on the surface of the planet. This has led to speculation that the first life may have emerged in deep-sea thermal vents with strong chemical gradients to energize biochemistry. The evidence for life has been captured in the geologic record and unsurprisingly is more ambiguous the farther back in time we go. There is circumstantial evidence for life in the ratio of carbon isotopes in outcroppings of rocks and in zircon crystals that are about four billion years old. Fossilized layered structures date back to about 3.2 billion years ago and are reminiscent of stromatolite mats that are abundant today. Microfossils that are 2 – 3 billion years old and have structures that appear to be imprints of microbial life. After the Cambrian explosion 540 million years ago, the geologic record becomes clear, recording the rise and fall of at different spatial depths that correspond to different look-back times.