14 Solar System Formation
Much of astrobiology is motivated by a desire to understand the origin of things: to find at least partial answers to age-old questions of where the universe, the Sun, planets, the first life on Earth, and we ourselves came from. On Earth, chemicals on the early surface at some point made the transition from non-living to living — the process of abiogenesis. We can take a step back and ask how the solar system formed and why the terrestrial and jovian planets ended up with their initial compositions. Then we can see if the model for how our solar system formed can describe exoplanetary systems as well.
Learning Objectives
By the end of this chapter, you will be able to:
- Explain how stars are formed in giant molecular clouds.
- List the main properties of the planets in our solar system.
- Describe the main steps in forming the solar nebula.
- Discuss how the solar nebula theory explains all of the properties observed in our solar system
- Discuss evidence that supports the nebular theory for forming solar systems.
Star Formation
At the heart of our solar system is a star. Stars of any type can host a planetary system and the most common type of stars in our galaxy are small, cool red dwarfs. The ultimate fate of a star, from birth to death, are determined by its initial mass. Where does this mass of material that forms a star come from? The answer is from huge clouds of gas and dust called Giant Molecular Clouds (GMCs).
Spiral galaxies like the Milky Way contain about 1000 GMCs, most located in the spiral arms of the galaxy. GMCs are among the largest objects in galaxies, with physical dimensions spanning 10 light years up to 1,000 light years. GMCs are cold enough for molecules to remain stable and are made of hydrogen (mostly H2), helium and other molecules as well as dust particles made of carbon, iron and silicates. All of the main building blocks for life — carbon, hydrogen, oxygen and nitrogen (CHONs) — were present in the cloud that collapsed to form our solar system. Ultimately, this concentration of gas and dust collapses to form hundreds of thousands of new stars and planets.
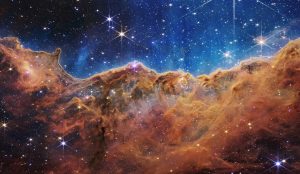
Side Note. Roughly half of the stars in our galaxy emerge from a GMC gravitationally bound to another star. Such stars are called “binary” stars and they can have orbital periods as short as an hour or as long as thousands of years. The O and B type stars are exceedingly rare. However, roughly 75% of these massive stars are gravitationally bound in binary star systems. In contrast, about 60% of stars like our Sun have a gravitationally-bound stellar buddy and fewer than 40% of the smallest stars – the low mass M dwarfs – have binary stellar companions.
A GMC is stable when the force of gravity, which tries to collapse the cloud, is balanced by the pressure from random motions of molecules in the cloud. Gravity is a force that pulls objects together and only depends on the mass of the objects and how far away from each other they are; the closer the objects, the stronger the gravity. A shock wave from a nearby star exploding as a supernova or some other event that compresses the density of the cloud can cause the cloud to collapse and fragment into smaller clumps. These smaller clumps are the seeds of solar systems, and it is the mass of these individual clumps that determines the fate of the star that forms at its center.
As a clump contracts, the density and the collision rate between particles increases so that the core of the cloud begins to heat up. At this point, the core of the cloud is a protostar with a temperature of a few thousand degrees, embedded in an obscuring shroud of cooler gas. As the clump contracts, it spins up and flattens and the object becomes a protoplanetary disk, or proplyd.
Want to know more – star formation in spiral arms of the Milky Way
It is interesting to map out the location of star formation. Stars are distributed nearly uniformly in the disks of spiral galaxies, but you would never know it looking at the composite image of the Whirlpool galaxy. The bright spiral arms are regions of enhanced density where new star formation is triggered. Because the massive stars are the most luminous, they light up the arms during their relatively short lives and are never seen far from the molecular clouds. The youngest stars are born in clusters located along the spiral arms of the galaxy and the giant molecular clouds are seen as dark dust lanes along the trailing edge of the spiral arms. The spiral density waves that cause turbulence and large scale coherent structure are observed only in flattened galaxies but the forcing mechanism that causes the spiral density waves is complicated and still controversial.
The Whirlpool Galaxy presents a view of dust lanes and massive stars tracing out spiral arms. Would you guess that there are more stars along the spiral arms? If so, you would be wrong… so what is going on? The spiral structure is a region of higher gas density, where bright, young stars are forming.
Solar System Observations
Any theory of solar system formation must be able to explain all of the properties of existing solar systems. This includes not only our solar system but the properties of exoplanetary systems in our galaxy. We will stay focused on first explaining the properties of our own solar system, via the solar nebula model. The video below was made when astronomers had only detected about 500 exoplanets (now there are more than 5000) and summarizes the properties of objects in our solar system.
Video credit: @NatGeo
After watching the video clip above or doing some research, make a list of similarities in the properties of the planets in our solar system. For example, do all planets orbit around the Sun in the same direction?
Now list any unusual patterns that you observe. Are comets and asteroids found at random distances or in specific locations?
Show Answer
- There are two types of planets: small, rocky planets (terrestrial) and large gas giants (jovians)
- Terrestrial planets are located in the inner part of the solar system; jovian planets in the outer part of the solar system
- Terrestrials are denser than jovians
- All of the planets revolve around the Sun in the same direction and the rotation (“spin”) of the Sun has the same direction as the orbital revolution of planets.
- The orbital planes of the planets are inclined by only a few degrees with respect to each other. In other words, planets all revolve around the Sun in approximately the plane of the Sun’s own rotation.
- All planets rotate on their axes with different tilts
- The solar system contains large numbers of asteroids and comets that are at specific distances
- All jovian planets have moons and rings; no terrestrial planets have rings and only Earth and Mars have moons.
We can organize the properties into three main categories: motion constraints, size and chemical constraints, and distance constraints. We call them constraints because they place restrictions on our theories; unless a theory can explain the observed facts, it will not survive in the competitive marketplace of ideas that characterizes the endeavor of science. Let’s take a look at these constraints one by one.
There are many regularities to the motions in the solar system. All eight planets revolve around the Sun in the same direction and approximately in the plane of the Sun’s own rotation (the blue orbits in Figure 3). If looking down at the solar system from a vantage point above it, this direction is counterclockwise. With the exception of the comets and other objects orbiting beyond Neptune, such as dwarf planets, the motions of the system members define a flattened disk or pancake shape.
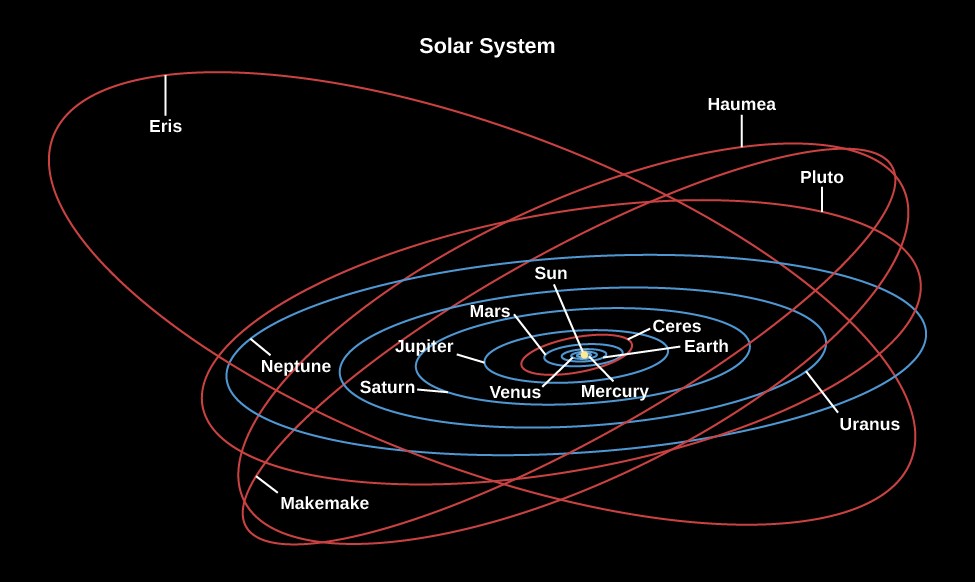
Side Note: Pluto was reclassified from a planet to a dwarf planet in 2006. The reason for Pluto’s “demotion” is that it has not cleared the neighborhood around its orbit, meaning it is orbiting the Sun with a lot of other material in the Kuiper Belt.
Most of the planets rotate in the same direction as they revolve, with the exception of Venus which rotates clockwise. Some planets have no tilt to their rotation axes while other are tilted at seemingly random angles: the Earth’s axis is tilted by 23.5°, for example. Figure 4 summarizes the different tilts.
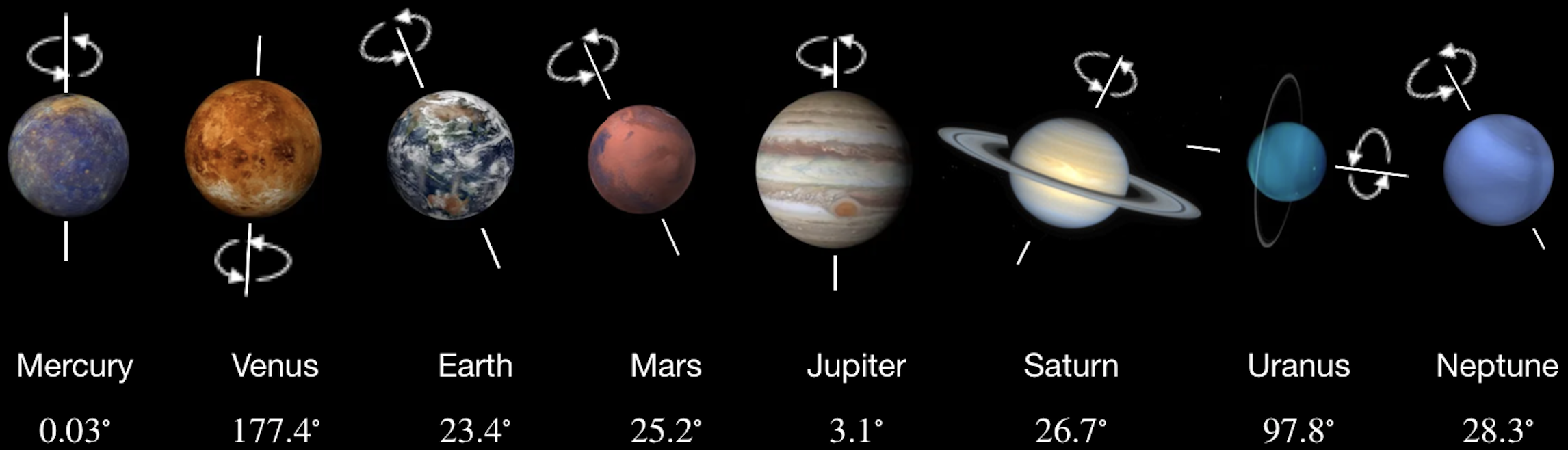
There are two main types of planets: rocky terrestrials (Mercury, Venus, Earth and Mars) and gas giants (Jupiter, Saturn, Uranus and Neptune). The terrestrials are smaller in both mass and radius than the jovians. By chemical constraints, we mean that the different types of objects in the solar system — terrestrial planets, jovian planets, asteroids, and comets — all have different chemical compositions. The four terrestrial planets are rocky worlds with a solid crust made of rocks containing mostly silicate minerals (e.g., granite and slate). Although the jovian planets have rocky cores, they are vastly dominated by the very light gases hydrogen (H) and helium (He). These chemical differences are reflected in the relatively high densities of the terrestrial planets compared with the jovians. There are sub-trends within the trends as well that need to be explained. For example, the Moon and Earth have some similarities in their chemical compositions but some differences, too.
The terrestrial and jovian planets are at different locations in the solar system: the terrestrial planets are in the inner solar system while the jovians are in the outer solar system. In general, asteroids are found in a belt in between Mars and Jupiter, and comets are found out past Neptune in the Kuiper Belt.
In the next section, we describe the solar nebular theory for how our solar system formed, and explain how each of the constraints described above are successfully explained by this theory.
The Solar Nebula Model
The cloud of gas and dust that collapsed to became our solar system is called the solar nebula. Our solar system was formed from this cloud beginning 4.6 billion years ago. The figure below shows an artist’s sketch of material in the solar nebula orbiting the protosun: many collisions occurred during this time and eventually material clumped together into larger objects: gas and dust grains stick together via first electrostatic forces and then gravity to form clumps, these clumps accrete more material through their increased gravitational pull and become planetesimals, and these planetesimals further grew to become protoplanets and eventually planets.
As the solar nebula collapsed under its own gravity, material fell toward the center, where things became more and more concentrated and hot. Increasing temperatures in the shrinking nebula vaporized most of the solid material that was originally present. At the same time, the collapsing nebula began to rotate faster through the conservation of angular momentum, which tells us that a rotating object will spin faster as it gets smaller (or spin more slowly as it gets larger). Like a figure skater pulling their arms in to spin faster, the shrinking cloud spun more quickly as time went on. Now, think about how a round object spins. Close to the poles, the spin rate is slow, and it gets faster as you get closer to the equator. In the same way, near the poles of the nebula, where orbits were slow, the nebular material fell directly into the center. Faster moving material, on the other hand, collapsed into a flat disk revolving around the central object (Figure 6).
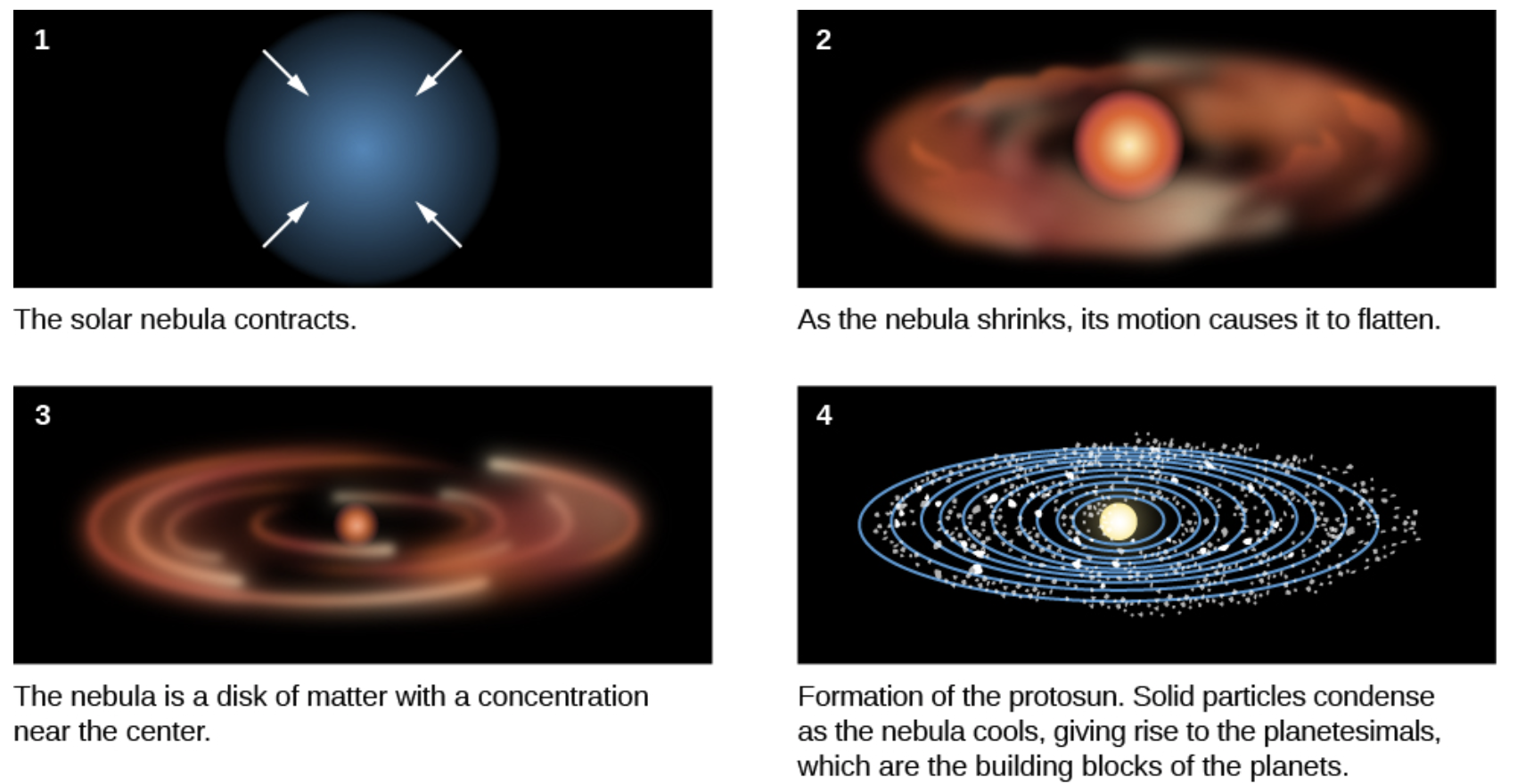
It eventually gets hot enough at the center of the disk (10 million K) for the protosun to begin fusing hydrogen into helium; this marks the beginning of the Sun’s life as a star. In the last stage (4) shown in Figure 6, material is clumping together within the lanes that formed in the solar nebula, and planetesimals accrete more material to become protoplanets and then the planets.
How do we know?
Protoplanetary disks had long been theorized to exist in star forming regions and evidence of their existence began with the detection of a circumstellar disk around the star β Pictoris in 1984 with the Infrared Astronomy Satellite. The images of the protoplanetary disks in the Orion Nebula in the figure below were taken with the Hubble Space Telescope (HST), which launched in 1990. The bright points at the center of the proplyds in Figure 7 are young protostars that are surrounded by dusty disks. Many other proplyds were observed in the Orion Nebula by the same team using HST, and this was a very significant finding in 1992. This is the same year that the first exoplanet was detected orbiting a pulsar and there was still much speculation on how common other solar systems were in our galaxy. Finding a large number of proplyds in just one nearby star forming region strongly suggested that the process of planet formation could be common, and perhaps other planets could host life.
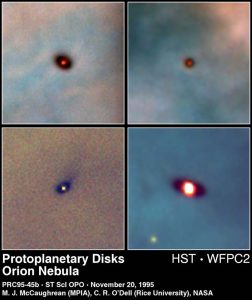
The temperature within the disk decreases with increasing distance from the Sun, much as the planets’ temperatures vary with position today. As the disk cooled, the gases interacted chemically to produce compounds; eventually these compounds condensed into liquid droplets or solid grains. This is similar to the process by which raindrops on Earth condense from moist air as it rises over a mountain.
Closer to the disk, it was too hot for hydrogen compounds to solidify into ices; at these higher temperatures, only rocks and metals could condense into solids. Eventually, at a certain distance away from the star, it is cool enough for the hydrogen compounds to also condense into ice. This idea is shown in Figure 8 below, where the line beyond which it is cold enough for hydrogen compounds to freeze is indicated as the frost line or snow line. The location of the frost line depends on the mass of the star that is forming at the center. For a star like the Sun, the frost line is 2.7 AU from the center. It will be closer for a low mass star and further our for a more massive star.
This very intuitive idea — that temperature decreases as you get further from the star — goes a long way in explaining the chemical differences as well as size and density differences between the terrestrial and jovian planets. The jovian planets are much more massive than the terrestrial planets, while the terrestrial planets have a higher density than the jovians. The terrestrial planets are rocky and have average densities of 5.0 g/cm3, while the jovian planets have an average density of 1.2 g/cm3 (for reference, at STP, hydrogen gas has a density of 0.089 g/cm3, water has a density of 1.0 g/cm3, silicon has a density of 2.3 g/cm3, and iron has a density of 7.9 g/cm3).
Let’s start with the mass differences. The terrestrial planets are made of rock and metal, and the jovians are made of rock, metal, and ices. Ice has mass, so the jovians grew more massive as they accumulated ice on top of the rock. The higher mass from the ice meant that gravity was stronger for these planets and hence more material could be pulled in. More material means more mass and stronger gravity…this self-reenforcing process continued until the jovians had pulled in all of the hydrogen and helium gas. This explains the mass differences.
As for density, since the additional ice and gases that the jovians pulled in have very low densities, this brought the overall densities of the jovians down to values that are much lower than rock. The density of Jupiter is 1.3 g/cm3 and this is the average density for the entire planet. While Jupiter has a dense metal core, the planet is vastly dominated by the light gases helium and hydrogen so the average density is much lower than the values for metals and rock. Without these additional ice and gas layers, the terrestrial densities remain higher, with values matching those of rocks and metals.
The solar nebula model explains why planets should be expected to orbit in the same direction as the spin of the star: the planets and star all formed from the same spinning disk of material. Therefore, the Sun rotates in the same direction as the spinning disk and the planets all orbit the Sun in the same direction. The planetary orbits would also stay in the plane of the flattened disk, which explains why the orbits of the planets are coplanar (recall Figure 3).
The different locations of the terrestrials and jovians are also explained by the temperature in the disk where they formed. Asteroids and comets are left over planetesimals that were not swept up into a planet. Their compositions are also related to the conditions where they formed in the disk. Asteroids formed inside the frost line and are made of rocky materials. Comets formed outside the frost line, and are consequently rocks covered in a layer of ice.
The unusual features that we observe, such as the sideways tilt of Uranus or Venus’ upside-down rotation, can be explained by the solar nebula model as the result of collisions during the planet-building phase. What about all the moons and rings around the jovians? This relates back to the high masses of the jovian planets. Since these planets are so massive, their strong gravity pulls in some of the dust and gas from the solar nebula, thus becoming “mini solar nebulas” as they form. This swirling disk of material around the jovians is the material that forms their moons and rings. The terrestrial planets were not massive enough to pull in material from the solar nebula, so the terrestrial moons are thought to be the result of early collisions with planetesimals. Our Moon, for example, is believed to have formed from the debris of a collision between the early Earth and another large body (Figure 9). Evidence to support this formation scenario for the Moon comes from lunar rocks brought back to Earth by astronauts on the Apollo missions between 1969 and 1972. These rocks confirmed that the composition of the Moon is similar to that of the Earth’s crust and mantle.
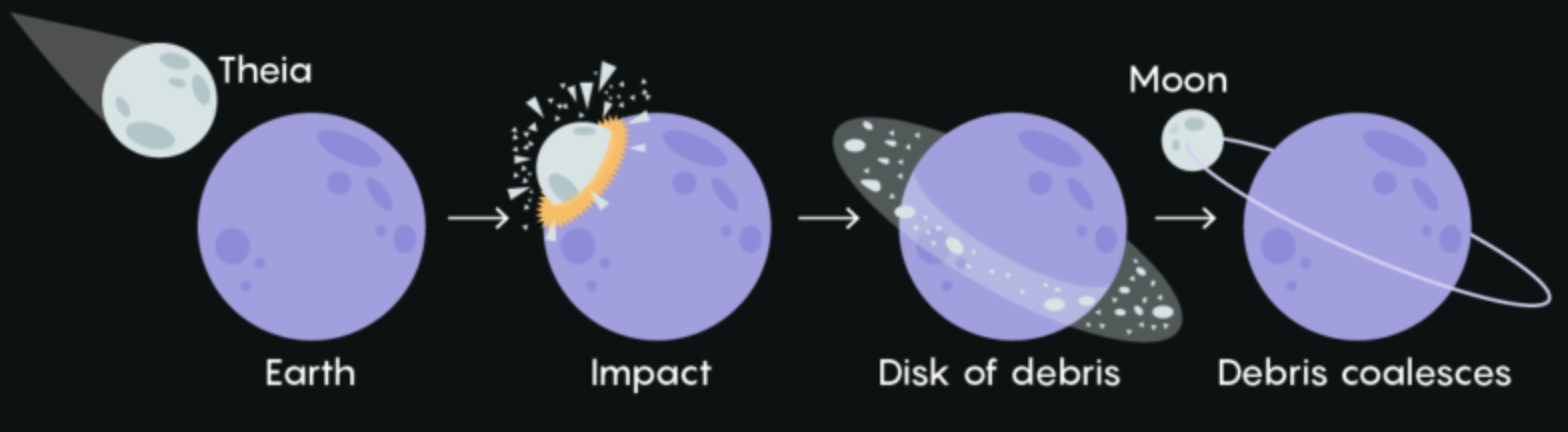
How do we know?
Dramatic evidence supporting the separation of material into distinct lanes or rings within the solar nebula came from this image shown to the right (Figure 10), which was taken in 2014 with the Atacama Large Millimeter Array (ALMA) of radio telescopes in the Chilean desert. This picture of the young star HL Tauri looks like an artists’s sketch of a solar system forming; in fact, it looks quite similar to how panel 3 of Figure 6 would look if sketched face-on rather than edge-on.
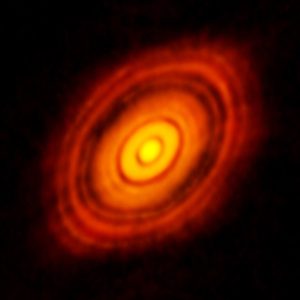
Comparison with other solar systems
Over 4,000 exoplanetary systems have been detected to date. Do they resemble our solar system, with four inner rocky planets and four outer gas giants. In a word, No. The animation below shows what the multi-planet systems detected by the Kepler Mission look like. Only the planets are shown and not the host stars and the planets are color-coded according to their temperature: the hottest “lava” planets are red and comparatively cooler planets, like Earth, Mars, and the gas giants, are colored blue. Planets that are analogues of Venus, with its hot rocky surface that could melt lead, are colored white. Watch the animation a few times and pause it to really take in how different all of these planetary systems are from our own.
Animation of multi-planet systems.
Video credits: Ethan Kruse/NASA Goddard, CC BY 3.0
There are some biases for the planetary systems shown in this animation, as the transit method that Kepler uses to detect planets will preferentially find planets that are orbiting close to their host star. We can still make the general conclusion that solar systems show an immense amount of variation. As we explore in Exoplanets: Statistics and Discoveries, the vast majority of exoplanets detected to date are different from the terrestrial and jovian planets in our solar system. In some cases, gas giants like Jupiter are found orbiting extremely close to their host stars. These “hot Jupiters” seem inconsistent with a formation scenario in which they formed so close to their stars. Computer simulations are being used to explore if perhaps these planets formed where expected but somehow migrated closer in to their star.
From the evidence we see of proplyds in our galaxy, we have confidence that the main idea of the solar nebula model — that solar systems form when a cloud of gas and dust collapses and forms a swirling disk of material with a protostar at its center — is correct.
Solar System Stability
Planets are kept in their orbits primarily through gravitational interactions between the planet and their host star. However, planets also feel a much smaller but non-negligible gravitational pull from the other planets in the system. Though the planets in our Solar System are relatively well separated, this is not always the case in other Solar Systems, and may not even always be the case for our own system. The closer two bodies are to each other, the stronger their gravitational pull will be on each other.
Close encounters between planets can affect their orbits’ eccentricities, semi-major axes, and relative inclination. These effects are accentuated in the case of resonant systems. Resonance describes the situation in which two planets have periods that form an integer ratio. For example, if one planet has a period of two years and a second planet has a period of four years, these two planets are said to be in resonance. Today, we can use computer simulations to determine what will happen to different systems of planets as they evolve through time that will expose these types of interactions.
Planets may also move around during formation. This may push smaller, more-likely rocky planets into the host star, or send debris like asteroids and comets into the path of other planets. Such motion in our Solar System is thought to have caused the period of late heavy bombardment, which left its mark on our Moon. This type of mechanism may have caused the strange position of hot Jupiters, which are commonly found and much closer in than where they could have formed.

In 2008, a paper by Batygin and Laughlin showed that on million-year time scales, planetary orbits may evolve in random, but ultimately stable ways. However, on longer timescales, orbits can rapidly and spontaneously evolve into chaotic states hard for even the best computer simulations to predict. Subtle interactions between the different planets and objects with mass in the solar system are capable of enacting significant change over the dynamical state of a system of planets. Close encounters will amplify these changes, rearrange orbits, and eject small bodies out of the Solar System. Resonant affects could even depopulate entire areas in a planetary system. The architecture and stability of a planetary system are important contributors to a planet’s potential habitability.
Key Concepts and Summary
Stars form when high density regions in giant molecular clouds begin to gravitationally collapse. As the protostar is collapsing, conservation of angular momentum forces it to spin faster and a disk of gas and dust settles around the equator of the star. This is the protoplanetary disk, where clumps of material collide and grow to form planetesimals, which collide and grow to form planets. In our solar system, there are two types of planets that formed: smaller rocky planets with thin atmospheres and gas giant planets. The solar nebula model describes formation of the solar system and describes the main features that we observe: the rocky planets orbit more closely to the Sun and gas giants formed and orbit beyond the ice line. The process of planet formation takes almost a million years, far beyond the lifetime of human astronomers. However, the discovery of young stars with protoplanetary disks and the structure of exoplanetary systems have helped to confirm and extend our understanding of star and planet formation.
Review Questions
Summary Questions
- What are giant molecular clouds? How do star systems form within GMCs?
- List the main observed properties of the planets in our solar system.
- What is the solar nebular theory?
- What properties are different for terrestrial and jovian planets?
- How does the solar nebular theory explain each of the properties observed in our solar system?
- How do comets and asteroids differ from planets? Where are comets and asteroids found in our solar system?
- What evidence do we have that the solar nebula model explains the formation of other solar systems in our galaxy?
- How did Earth’s moon form? What evidence supports this claim?
- Do other solar systems have the same layout as our own? What evidence supports your answer?
Exercises
related to or belonging to the Earth. For exoplanets, terrestrial planets are rocky worlds, composed of rock, silicate, water and/or carbon.
related to or belonging to Jupiter; jovian worlds are gas giant planets, similar to Jupiter
Objects in our local planetary system that are gravitationally bound, including the Sun, the eight planets and their moons, asteroids, comets, and other debris.
stars that are similar to or less massive than the Sun.
giant molecular clouds are cool clouds of dust and gas that seed the formation of thousands of stars.
pressure wave created by a powerful explosion
an early stage for a high density clump of material that will form into a star
a disk of gas and dust where planets will form
planetary systems orbiting other stars