27 Speculations and Skepticism
Here in the last chapter of our textbook, we ask you to consider all that you have learned about the scientific investigations seeking to discover life beyond the Earth. So far, we have not been successful in discovering such life, but hopefully in the near future we will find some evidence. In the meantime, a large contingent of people have argued that they know that life forms from beyond the Earth exist and that they have actually visited us. Some of these people have been prominent individuals with a lot of resources at their disposal to make their claims seem exciting and more convincing than they actually may be. However, these claims have not stood up to close scrutiny. There are very few claims that have made it past any sort of peer review, and those few peer reviewed papers which have made bold claims to have discovered evidence for life beyond the Earth have ended up criticized, contradicted, and, in some cases, retracted from publication by the rest of the community. Nevertheless, the popular media continues to promote many of these ideas that there are not accepted by the scientific community. In this chapter, we will explore these ideas, try to decide how to distinguish science from pseudoscience.
Learning Objectives
By the end of this chapter, you will be able to:
- Critically assess claims made in various locations, especially in the popular media, by use of Carl Sagan’s baloney detection kit.
- Explain various natural phenomena, human technologies, hoaxes, and delusions that have been called “UFOs”.
- Discuss how some beliefs, especially those related to religion and spirituality, form part of some people’s arguments that life beyond the Earth exists and interacts with us.
Since we have yet to find scientific evidence for life outside of the Earth, much of the study of astrobiology is necessarily speculative. Perhaps because of this, there has been and continues to be a tendency for people to believe that evidence does exist for life from beyond Earth interacting with us.
This tendency can be seen in the mythologies associated with the skies across many human cultures. Intelligence and life was assumed to be part of the celestial world, with gods, monsters, and the spirits of once-living terrestrial creatures complete with agency and personalities that could interact with humanity. Constellations, planets, and stars were the first “extraterrestrial life forms” that human beings assumed existed.
With an adoption of scientific perspectives on how the natural world operates, such enchantment of the natural world was eschewed. The planets, stars, and other phenomena we observe with our telescopes have up to now exhibited no signs of magical action, intelligence, or life. However, a somewhat large contingent of humanity takes issue with this lack of evidence and proposes, contrariwise, that there is in fact ample evidence for such. This chapter aims to examine some of these claims in some detail.
Before proceeding much further, however, the evidence that will be considered in this chapter will be, in most cases, rejected as problematic, incomplete, or even completely incorrect. How does anyone decide this is the case? Ultimately, you have to use your own judgment to weigh evidence, and this includes the scientific evidence that has thus far been presented in this book.
Skepticism
One possible approach to evaluating claims is that of skepticism. Skeptics, as those who adhere to this position are called, adopt a set of standards for evidence used to hopefully distinguish fact from fantasy. The nature of this position is, however, that there will be people who disagree with the skeptical perspective. If one person argues that a phenomenon exists, the skeptical position is to propose that unless there is convincing and verifiable evidence that can be measured, the phenomenon is assumed not to exist. This type of assumption is called the null hypothesis.
What standards of evidence would be enough to have a skeptic reject the null hypothesis? There is no firm demarcation, and this is likely why there is often controversy surrounding these subjects especially within the popular, philosophical, and sociological discourse. Nevertheless, surveys of experts and scientists tend to show remarkable agreement as to the lack of evidence for a lot of the ideas we will be discussing, according to some general principles of “skepticism” including a famous dictum often attributed to the late Carl Sagan, “Extraordinary claims require extraordinary evidence”. What follows is one possible set of criteria that attempt to offer a means to determine which claims are supported by evidence provided by the late Carl Sagan.
Baloney Detector Kit
In his 1991 book, Demon-Haunted World, Sagan attempts to provide his readers with a set of tools that can be used to indicate concern with certain arguments. These tools are not meant to be foolproof methods to determine with absolute certainty whether a claim is true or false, but the hope is as one starts to evaluate more and more claims, the “Baloney Detector Kit” will work to help steer the user in the direction of being able to identify which claims are worthy of more careful consideration and which can be dismissed more quickly. Entertaining every claim is an exhausting endeavor, and with limited time such techniques can be used to move on to more productive avenues of investigation. Sagan writes that the tools in this kit are the following principles, quoted here in full:
- Wherever possible there must be independent confirmation of the “facts.”
- Encourage substantive debate on the evidence by knowledgeable proponents of all points of view.
- Arguments from authority carry little weight — “authorities” have made mistakes in the past. They will do so again in the future. Perhaps a better way to say it is that in science there are no authorities; at most, there are experts.
- Spin more than one hypothesis. If there’s something to be explained, think of all the different ways in which it could be explained. Then think of tests by which you might systematically disprove each of the alternatives. What survives, the hypothesis that resists disproof in this Darwinian selection among “multiple working hypotheses,” has a much better chance of being the right answer than if you had simply run with the first idea that caught your fancy.
- Try not to get overly attached to a hypothesis just because it’s yours. It’s only a way station in the pursuit of knowledge. Ask yourself why you like the idea. Compare it fairly with the alternatives. See if you can find reasons for rejecting it. If you don’t, others will.
- Quantify. If whatever it is you’re explaining has some measure, some numerical quantity attached to it, you’ll be much better able to discriminate among competing hypotheses. What is vague and qualitative is open to many explanations. Of course there are truths to be sought in the many qualitative issues we are obliged to confront, but finding them is more challenging.
- If there’s a chain of argument, every link in the chain must work (including the premise) — not just most of them.
- Occam’s Razor. This convenient rule-of-thumb urges us when faced with two hypotheses that explain the data equally well to choose the simpler.
- Always ask whether the hypothesis can be, at least in principle, falsified. Propositions that are untestable, unfalsifiable are not worth much. Consider the grand idea that our Universe and everything in it is just an elementary particle — an electron, say — in a much bigger Cosmos. But if we can never acquire information from outside our Universe, is not the idea incapable of disproof? You must be able to check assertions out. Inveterate skeptics must be given the chance to follow your reasoning, to duplicate your experiments and see if they get the same result.
In the discussions that follow, try to see where these tools can be applied to the evidence that is provided. It is important to understand that these tools do not say “yes” or “no” in answer to the question, “Is this claimed phenomenon real or not?” The hope is rather to provide enough tools so that the merits of the claims can be evaluated and the reader can make up her or his own mind.
Unidentified Flying Objects
One of the first subjects that students are interested in exploring is the question of whether spacecraft from other intelligent groups are visiting Earth. An extensive catalog of stories attempting to demonstrate evidence for this has been assembled by various investigators often termed “ufologists” as a way of identifying that they study “UFOs”. The term “UFO” Is an acronym that was coined by Air Force Captain Edward J. Ruppelt in the 1950s to stand for the words “unidentified flying object”. At the time, this was intended to be a neutral indicator of the reports: most people who believe they have seen a UFO report that it is flying in the sky, that it is a physical object that can be seen or tracked by, for example, radar, and that the observer does not know what the object is making it “unidentified”. Over the decades, the term has become so closely associated with the claim that there are extraterrestrial intelligent beings visiting the Earth, that now many associate UFO with “alien spacecraft”.
The official investigations of UFOs first happened in a coordinated fashion after World War II. At the time, there was heightened interest in threats from flying objects of human origin. During the Second World War, aerial technology advanced quickly to jet-propelled aircraft and rocket artillery and in the United States a Civil Air Defense Corps was inaugurated to monitor the skies for incoming enemy threats. The culture of reporting unusual aerial phenomena to the authorities was well-established by the time UFO investigations sponsored by the United States Air Force first began.
Under a variety of monickers, after assembling a team of investigators, the US Air Force undertook a number of projects to investigate the reports and determine whether they could be explained or whether they constituted a threat to the national security of the United States. Over the course of nearly a decade, a number of reports came back indicating consistently that some sightings could be identified as being caused by known phenomena and the ones that could not be explained did not rise to a level such that they could conclusively point to any new phenomena beyond the prosaic explanations that identified the other sightings. Since there was nothing conclusive that could be ascertained from the reports the investigations were ended so as to avoid prolonging a wild goose chase.
Since that time, formal studies of UFOs have been only intermittently conducted by governments and the scientific community has all but ignored such ideas. Ongoing claims that evidence for UFOs exist continue to be made, but so far there has not been the acceptance that these extraordinary claims have generated any extraordinary evidence.

Popular interest in UFOs continues in part due to such stories featuring in entertainment and in journalism. H.G. Wells in 1897 wrote War of the Worlds which presented a scenario of intelligent lifeforms from Mars invading the Earth. Likely, Wells was influenced by the recently published book by Percival Lowell entitled, Mars where, erroneously, Lowell reported on the observations of Giovanni Schiparelli and others who argued that there was a network of canals that could be seen on the planet. This inspired considerable speculation that there could be flowing water and life on Mars, but later work revealed that these observations were entirely spurious.
At around the same time, humanity began experimenting with flight starting with airships that used hydrogen gas to achieve the necessary lift. A number of mystery airships were reported in the media with a few newspaper reporters uncritically claiming that they were made by lifeforms from other worlds who had used them to travel to Earth.
Reports of UFOs began to proliferate after a media sensation surrounding the claims of a pilot named Kenneth Arnold who in 1947 who reported seeing nine aircraft that appeared to him to move in a way that was far different than any aircraft he had seen until that point. He argued that such objects appeared to be moving like “saucers skipping across a pond” and the term “flying saucer” was soon attached to the unknown objects. On the basis of his personal observations, Arnold was convinced that aircraft were visiting Earth and were developed by an advanced extra-terrestrial intelligent beings. He consistently argued this for the rest of his life.
Not long after this, the now famous Roswell Incident occurred where a New Mexico rancher saw a balloon crash into a field and wreckage was recovered. The local news story complete with photographs and a visit by the officials from the Army Air Force who removed the physical evidence and eventually explained the event as a weather balloon crash although it was actually a nuclear testing monitoring balloon. The event might have disappeared into obscurity, but interest was revived in the late 1970s through the 1990s when a number of claimants began to argue that there had been recovery of extraterrestrial artifacts including lifeforms. The culmination of this sensation was the production of a so-called “Alien Autopsy” video which was broadcast nationally in 1995 and claimed at the time to be authentic footage, though the filmmaker years later would clarify that it was a reproduction of what he claimed to remember.

Identifying UFOs
Claims of visitations by intelligent lifeforms from beyond the Earth continue to be made in news stories, science fiction, and popular media. The very identification of a sighting of an “unidentified” object makes attribution difficult. The null hypothesis is that such observations have prosaic explanations and such explanations fall into four categories: natural phenomena, human technology, delusions, and hoaxes.
Natural Phenomena
A number of natural phenomena have confused observers into believing they were due to fantastical technology or the supernatural owing to peculiar characteristics, circumstances, or simply not knowing how the phenomena appears in nature.
A number of astronomical objects have been reported as “flying” in ways that observes thought looked like they were piloted by intelligent lifeforms, but this is not the case. Famously, the planet Venus is often puzzling for those who are unaware that it is the third brightest natural object in the sky and can rival the brightness of plane landing lights. In dark sites when there is no moon, Venus is bright enough to cast shadows. As a planet, it is also not a point source and can therefore be distinguished from stars because it exhibits less twinkling than stars exhibit as the random fluctuations in the atmosphere that cause twinkling stars are washed out over the larger apparent size of the planet. Venus is never far from the horizon, giving it the appearance of flying closer to the ground. When it is in the evening sky, Venus sets at approximately the rate of the Sun which makes it appear to be moving through the sky or perhaps landing at some distance. When Venus is very low in the sky, the same atmospheric refraction that causes sunsets can affect the color of Venus on short timescales, making it appear to be flashing different colored lights.
Some transient astronomical phenomena include meteors or bolides which appear as bright streaks and, in some instances, smoke trails in the sky. These are the visible signs that a rocky body from space has entered the Earth’s atmosphere on a collision course. Shooting stars can be seen almost any night, but some points during the year there are many more of them in so-called “meteor showers” at a point in the Earth’s orbit where it passes through the debris field of a comet. The largest fireballs are unusual, but can be extremely bright and happen high enough in the atmosphere to be visible for hundreds of kilometers. Occasionally, these meteors are large enough to cause damage when they hit the ground as meteorites or propagate shockwaves and sonic booms if they explode in the atmosphere.
Birds are, of course, flying objects and while most people think they can readily identify birds, some birds are large enough to confuse the perspective of the observer. One suggestion that has been made to explain Kenneth Arnold’s sighting was that he was observing a flock of pelicans. These large birds can fly fairly high and if they are not identified as birds, it is possible to misjudge their size. Arnold proposed that the objects he saw were the size of aircraft and that they moved very quickly. Pelicans are approximately the size of a small human and if they are mistaken for larger objects, the person seeing them may believe that they are farther away than they actually are as a large object can have the same angular size as a small object when it is at a further distance. What is more, a closer object will appear to move across the field of view at a faster rate than one that is further away, so it has been suggested that Arnold’s estimate of the speed of the objects could have been misjudged as being much faster if he assumed the objects were further away than they were.

Another instance of a possible bird sighting was the so-called “Flatwoods Monster” which was a reported contact with what the observers thought was an extraterrestrial lifeform. The night of the incident was the same night that a meteor was observed, and the people who reported seeing the Flatwoods Monster went to investigate the meteor crash in the local forest. When they happened upon the location where the meteorite hit, they reported seeing a human-like figure that scared them. A sketch artist’s impression of what they saw was mocked up, and a comparison of the drawing to what a barn owl on the top of a moderately-sized tree or stump has been suggested as an intriguing correlation.

A few other usual natural phenomena have been identified in connection to UFO reports. Will-o-the-wisp are claims of glowing lights typically seen in marshy or swampy areas that have identified as sometimes being due to the igniting of combustable methane gas. St. Elmo’s Fire has been described since antiquity but has only recently been identified as electrical discharge that can occur in certain situations where there is substantial charging in the atmosphere as what might happen before a thunderstorm.

Finally, cloud formations have been misidentified, especially lenticular clouds. These clouds are formed under very particular atmospheric conditions and look superficially like the stereotypical “Flying Saucer” spacecraft often claimed in UFO reports. When such clouds appear in the evening or morning, they can reflect and refract light from the sun on the horizon taking on unusual coloring and mimicking flashing lights.
Human Technology
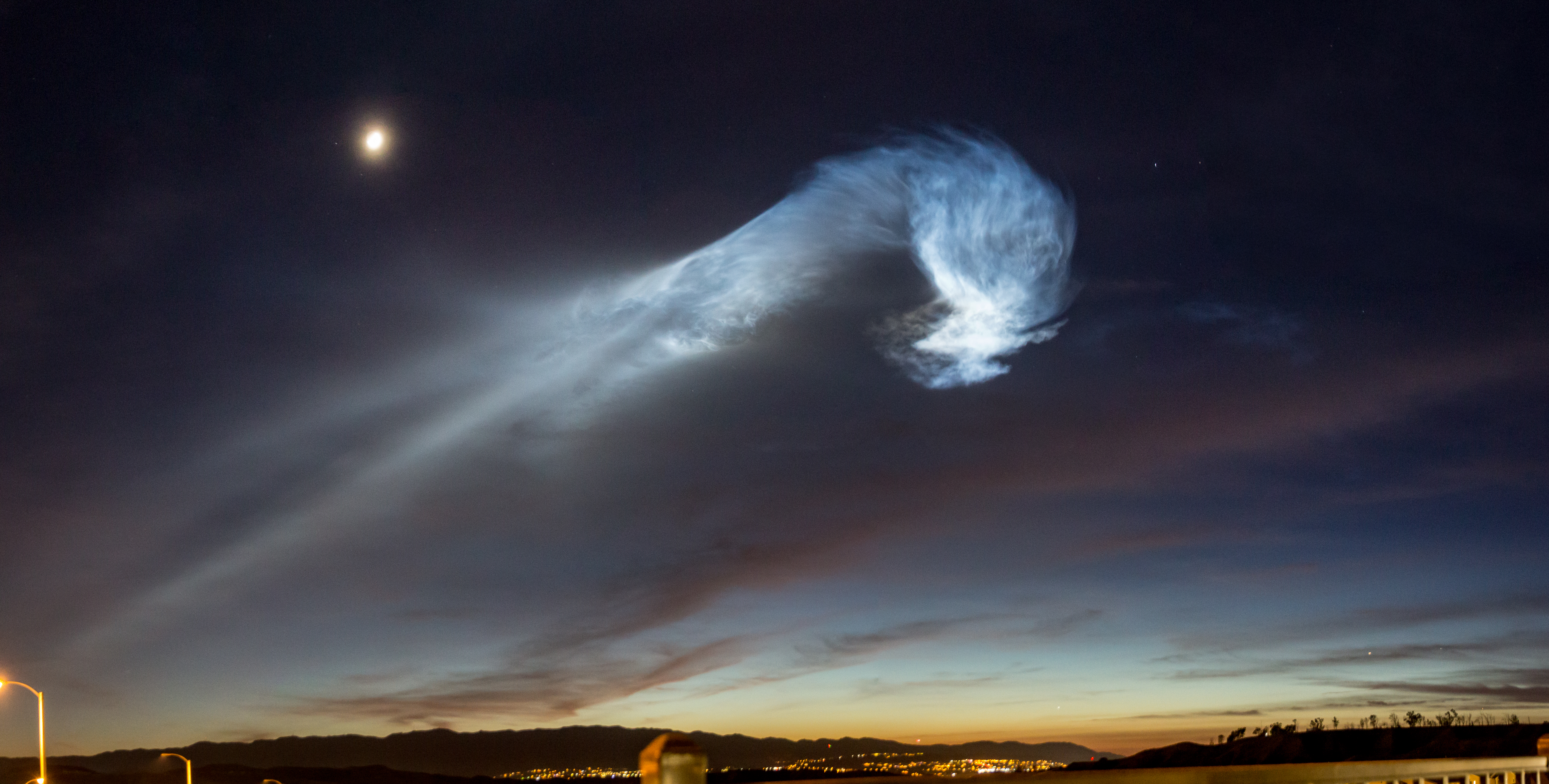
Many comparisons to UFOs are directly to human technology either as the airships of the late nineteenth and early twentieth centuries through to jet aircraft and rocket ships today. Even so, rocket launches are unusual enough that when they happen and an unsuspecting observer happens to be looking in the direction of a launch, they can be led to believe that they are witnessing something otherworldly. An example of this is the 2009 Norwegian Spiral Anomaly which formed a dramatic blue spiral visible from many parts of Norway one evening. It was identified as a failed rocket launch from Russia, but the unusualness of contrails and exhaust that come from the launch are such some classify it as a member of a class of “Space Jellyfish”.
Once in orbit, satellites can appear in ways that are very unusual in comparison to planes. Low Earth orbit implies a satellite that passes directly overhead will cross from horizon to horizon in 45 minutes, but most of that time will be spent near the horizon. As it passes overhead, it moves at a considerable clip crossing the distance of five full moons in one second. Such satellites reflect the light of the Sun and so can mostly be seen near sunrise or sunset. Low-Earth orbiting satellites pass either into or out of the shadow of the Earth appearing to appear or reappear suddenly, and if the architecture of the satellite is favorable, this can lead to satellites appearing to “flare” as they point a flat reflective surface towards the unsuspecting observer.
Perhaps one of the most famous UFO-related human technology observed are balloons. While most people have experience with party balloons, there are extremely large balloons used for scientific and military purposes which look nothing like those at a party. These high-altitude balloons do not fully inflate until they reach the height of the stratosphere past 15 km or so. Once at these heights, the balloons inflate to the size of a small building and in the early evening when they are typically launched will reflect the setting sun as they travel quickly through the action of the jet stream. West Texas and New Mexico are the launching point for much of the scientific ballooning done in the US and often reports of UFOs to the local media increase during the launch season.
In the last few decades, robotic drone flyers have become another source for confusion by people seeing unexplained things in the sky. Drones used for classified missions have confounded pilots as the size of the drones and therefore their distances are difficult to ascertain. Drones may also be subject to radar detection, but in the case of military uses, stealth technology can be employed to hide or confuse the radar signal so that distances and speeds cannot be accurately ascertained. A number of the latest UFO sightings breathlessly reported in even reputable media have been attributed to drones by skeptics. Commercially available drones are also one of the most common ways to create a UFO hoax.
Delusions
A certain number of UFO sightings are due to delusions, but it is important to realize that anyone can suffer from delusions. The accusation that someone is delusional is often a tool of derision, but intelligent, capable and otherwise competent human beings are suspect to any number of effects that can cause them to believe they have perceived something that they have not perceived. A common example of this is the so-called “Misinformation Effect” where memories can be distorted and inaccurately recalled due to the introduction of misleading information or suggestions. Indeed, suggestibility is a psychological phenomenon that is offered to explain how hypnosis functions. Studies of such effects have cast doubt on the reliability of eyewitness testimony in courts, and in instances where testimony is the only evidence for an observation of a UFO, such effects may be cause for concern. Unfortunately, those who offer eyewitness testimony are often completely unaware that their memory has been distorted and instead take umbrage at the suggestion that they are mistaken, but such concerns are not intended to cast those offering their testimony as liars. Instead, it is an effect to keep in mind as a possible explanation for a testimony that was intended to be offered truthfully.
In some instances, these sorts of misremembered events can be the result of trauma. An instance of this seems to be indicated in the community of abductees who claim with varying degrees of detail and intensity, that they have not only been visited by lifeforms from beyond Earth, but that these lifeforms have captured and, in some cases, tortured them. Some accounts follow patterns that have been identified by psychologists as similar to a condition known as sleep paralysis where a certain somnambulatory state between dreaming and waking happens when a person is aware of what is happening but is unable to physically move.

The first claimed abduction is worth recounting due to the iconic nature of its occurrence. Barney and Betty Hill were an interracial married couple living in New Hampshire in 1961 at a time when interracial marriages were uncommon and actually illegal in many parts of the United States. During a drive home, they saw a UFO, followed it in their car, left the vehicle to investigate, and then became very frightened of what danger it could pose. They reported a strange loss of time upon their arrival back home. For some days afterwards, they were haunted by the trauma of the event and Betty had reported troubling dreams. At the time, UFO stories were still being investigated by national authorities, and the stories often ended up reported in the press uncritically. Also at the time, hypnosis was being actively used in a way we now know is problematic: to help people recall so-called “repressed memories”. Unfortunately, it has been convincingly documented that encouraging people to participate in an activity where they are subject to suggestibility as in a hypnosis session is a way to plant false memories. Nevertheless, through hypnotic sessions that began some years after the incident, Barney and Betty Hill began to describe more remembered features of their encounter including an abduction by what they described as extraterrestrials who took them to their spacecraft and examined them. Betty Hill outlived her husband by decades and continued to produce more and more claims about her contact with the extraterrestrial lifeforms including describing the aliens as the now familiar “gray alien”, and eventually determining that they came from a planet orbiting the star Zeta Reticuli. An entire community of people believe that “Zetans” are the intelligent extraterrestrials visiting Earth largely based on Betty Hill’s prolific accounts that are stored in the archives of the University of New Hampshire library.
Delusions do not only happen to individuals. There are instances of so-called “mass delusions” where multiple independent people or groups of people become convinced of events or phenomena which simply do not exist. One example of this that predates the UFO phenomenon were reports of the Edison Star in March and April of 1897. At this time, there was concern across the United States that Thomas Edison was producing an artificial star to light up the night. Sightings of this star happened in many locations, but there was no such object to be seen. This event is very reminiscent of various “UFO waves” or “UFO flaps” which occur in places from time to time. As media interest intensifies, more and more reports are made of people seeing unexplained objects flying in the sky to the point where the argument is offered, sometimes even explicitly, that if it had to be interactions with extraterrestrial lifeforms because otherwise there would not be so many sightings. After an increasing flurry of interest, the reports eventually come to an end as the attention of the media and society is moved towards other things.
Hoaxes
Since the claims began to proliferate, hoaxes have been promulgated. Faked photographs, video footage, and physical evidence have been used to claim “clear proof” and discoveries of hoaxes inevitably lead skeptics to question claims that come after this. One instance where a hoax was strongly suspected was the first photograph ever taken of a UFO from a farm near McMinnville, Oregon. The pair of pictures appear to show a flying saucer and the claim was that the pictures also indicate the UFO was moving from one part of the scene to the other. Suggestions that the photographs were faked were made almost from when they were first published, most explanations proposing that either an old motor, a hubcap, or a dinner plate was suspended by fishing line. The family never admitted that it was a hoax.

Perhaps the most famous hoax associated with ufology was that of crop circles. For years in the late 1980s and early 1990s, crops in south England were discovered mysteriously flattened in circular patterns that became increasingly elaborate. A craze of investigation happened that captured worldwide attention. Various phenomena were invoked as plausible explanations including claims by many who believed that UFOs were extraterrestrial spacecraft that the crop circles were related to the intelligent lifeforms. In spite of the media sensation, in 1991 two men admitted to producing most of the crop circles in England using fairly rudimentary devices. Even as they were able to demonstrate their production of crop circles, there still remained believers convinced that this was not the explanation some of whom still do not believe the phenomenon was a hoax. Crop circles continue to be produced by artists and other aficionados including one that famously paid homage to the Arecibo Message.
An idea that just will not quit
In the last ten years, in the United States, a newfound resurgence in belief in UFOs as being evidence for life from beyond the Earth has taken hold. In 2023, congressional hearings were held where certain former members of the Department of Defense argued that there was a lot of evidence being withheld that confirmed the existence of intelligent life forms that were “non-human”. The evidence for this, they contended, was not possible to provide directly because it had been classified or was being otherwise suppressed. A student equipped with Sagan’s baloney detection kit should pause right there. When evidence is not able to be examined or considered, it is difficult to come to any conclusions.
At the same time, some videos were released that had been captured by sophisticated military cameras purporting to show things flying in strange ways. However, for each video, skeptics have provided alternate explanations that do not require anything beyond natural phenomena, human technology, hoaxes, or delusions. In some cases, it seems that equipment malfunction or miscalibration could be an issue. In other instances, trained pilots may have been misled by optical illusions or known natural phenomena or human technology.
Some of the people who have seen strange things in the sky were sometimes embarrassed to report their observations. In fact, fear of ridicule may be exactly why some of reports were not made or were made less than carefully, making it more difficult for investigators to identify what was being reported. Since human technology, after all, is one of the categories that UFOs have been identified as, there were some professionals worried that uncrewed drones or balloons could be an explanation for some of the sightings, and this technology could be made by people or governments with malicious or spying intent. Determining that this was the case would require better documentation and observations than what might have been happening because of embarrassment.
What if you see something in the sky you cannot explain or understand and capture a video of it with a personal device? Mick West, a skeptic who maintains a keen interest in these sorts of questions, recommends that you do a little more than just post the video to social media. After all, the video by itself can leave more questions than answers since it may not be clear how it was taken or what the conditions were. The most crucial information you should make sure to document are the so-called “details” the DTLs: date, time, and location. A surprising number of UFO videos online lack these basic pieces of information. And, unfortunately, hoaxes are just as rampant today as they ever have been. With the “DTLs” it becomes easier to identify what exactly is being shown in a video.
The hype and excitement have not died all the way down at the time of the writing, even as there remains no strong evidence for any of these sightings requiring explanations that go beyond the four we give you in this chapter. Some famous politicians, musicians, soldiers, and even a few scientists and professors have supported some of the claims, but the scientific community has been fairly steadfast in rejecting those arguments. In one instance, a committee convened by NASA spent more than a year studying the issue and showed that for the UFO (styled “UAP” or “unidentified aerial phenomena” in the report) sightings where the data is of the highest quality, it is possible to show that there are mundane explanations do not require life beyond the Earth. You can read the report for yourself!
In sum, in spite of the lack of science, there are a lot of people who are convinced beyond any doubt that there are UFOs which were created by life beyond the Earth. For some of these people, there is no amount of argument, explanation, or skepticism that will convince them otherwise. However, equipped with our baloney detection kit, we are at least given some means to pause and think. Science demands that we ask questions, so when a UFO believer argued that there are no questions that can be asked to show them wrong, this may indicate that the evidence is not all they are cracking it up to be.
Conspiracy Theories
Even with the large number of prosaic explanations for claimed UFOs and the number of reports issued that concluded there was no strong evidence for UFOs indicating an interaction with life from beyond the Earth, there continue to be those who believe that some UFOs are not explainable by the four categories of identifications listed above. When faced with the question as to why government agencies and experts dismiss such arguments, there is sometimes a claim that there is a conspiracy among a large number of interested parties to conceal the truth of the matter. Stories as to how this has done often include hiding evidence at secret military installations such as the famous “Area 51” in the United States Air Force’s Nevada Test and Training Range where the promoters of the “Alien Autopsy” video claimed evidence was being hidden. There are declassified documents which indicate that the United States Armed Forces in part did not spend time refuting such claims in order to hide the true classified nature of some of these institutions (some of which were involved in the production of espionage technology, for example).
Whether these stories are promoted by people who know they are incorrect or whether they were promoted by people who truly believe in “alternative scenarios” is hard to determine. Suffice to say, these expansive narratives become increasingly complicated as the interested interlocutor continues to ask additional questions about the existence or lack of existence of evidence for such conspiracy theories. Inevitably, completely unrelated arguments end up incorporated. Arguments get made such as, “If NASA is lying about the existence of extraterrestrial lifeforms interacting with human beings, perhaps it is also lying about the shape of the Earth.” Aside from the fact that it is possible to perform some simple experiments yourself to discover that NASA is not lying about the shape of the Earth, the chain of argument becomes longer and longer and weaker and weaker as the explanations continue.
One particular conspiracy theory that has gained traction in the popular media is the proposal that not only are extraterrestrial lifeforms interacting with Earth, but that there is evidence that they have been doing so for millennia. The evidence for such “Ancient Aliens” is usually the large structures that humans built before the Industrial Era. The pyramids of Egypt and Central America, the megaliths of Europe and Asia, the now-abandoned cities of the past in the Eastern and Western Hemispheres are all cited as in certain television shows and popular books that there must have been contact with intelligent lifeforms possessing advanced technology to enable humanity to construct these impressive structures.
Archeologists and anthropologists have entire journals dedicated to explaining the way in which our predecessors constructed the large and complicated features of their societies. Not everything is completely understood, but there is ample evidence of the construction techniques, design knowledge, and abilities of the craftspeople from written records, artifacts, and even oral histories. The presumption by many of those arguing that human beings were incapable of achieving the incredible feats of the past is that because they do not understand how, for example, a pyramid could be built therefore no human being can. The people who built the pyramids beg to differ.
The allure of such arguments is that they excite the imaginations of many. In the modern world, science fiction and fantasy literature may hold functions similar to those that folktales and mythologies used to hold. That some people confuse these stories for factual accounts is not surprising, but as with any claim to truth, it is important to keep stock of the full range of possibilities and narrow on in explanations that are more likely to be correct while avoiding those that are more likely to be incorrect. Hopefully, the baloney detector kit can work.
An Enchanted Universe
The connection of these ideas to mechanisms that are unknown to scientific investigation inspires some people to delve into open questions which have been attempted to be answered through alternative philosophical and religious means. Examples include: Is there something special about life that requires explanations that our physical models will be unable to provide? Are there ways that we and other aspects of reality are influenced beyond the four forces of physics?
While open questions of this sort are, by definition, not answered, there are auxiliary proposals and ideas for which we can examine the evidence.
Astrology
The predictability of the motions of the heavens enabled early astronomers to become accurate timekeepers and calendar makers. This skill required observation and modeling as we currently enjoy, but there were extensions to such predictions that were abandoned by the scientific community in the eighteenth century. These included so-called “astrological” claims that the planets influenced human affairs and current events through personality-like influences. These claims continue to be made today with the influences argued as being due to personal characteristics that the planets and constellations were given. For example, Mars was considered a masculine god of war and Venus a feminine goddess of love. The position of these visible planets with respect to the Sun, Moon, and background stars was meant to influence daily life in individuals depending most strongly on where the Sun appeared to be when they were born, and less strongly on where the Moon and the rest of the planets were. As the Sun, Moon, and planets all orbit in a plane, the only constellations that they can be seen in are the twelve constellations that form the Zodiac or the Plane of the Ecliptic which indicates the orientation of the plane of our Solar System with respect to the Earth (if there were no tilt to our axis, the Plane of the Ecliptic would align with the Celestial Equator).
The idea that the position of the planets with respect to stars influences human behavior or future outcomes generally was (and, by contemporary believers in astrologers, continues to be) attributed to the same mysterious forces which kept the planets moving in their courses. However, with the advent of both the heliocentric model for the solar system and our modern theory of gravity, it became possible to explain the motion of the planets and precisely calculate the strength of the forces that planets exert. The scientific explanations for the motions of the planets often recast the stories of astrology in a different light. For example, retrograde motion of the planets still worries people, many of whom do not know what retrograde motion is. At various points along the synodic period of a planet (the time it takes for a planet to return to the same position in the sky), a planet will appear to stop moving in the normal direction it wanders across the constellations of the Zodiac. At that point it will turn apparently make an abrupt change of course and move in the opposite direction before stopping again and returning to its previous path. This retrograde motion is said by astrologers to indicate a disorienting or disordered characteristic to the planets’ motion (recall that planets were once considered imbued with consciousness as deities or spirits), but in fact it is the result of the Earth moving around the Sun. The analogy can be drawn to cars passing each other on a roadway while traveling in the same direction. For most of the journey, the cars will appear to move in the same direction, but at certain points one car will pass another causing the appearance of the cars moving in different directions. Retrograde motion occurs as the Earth passes the planets that are farther away than the Sun or as the planets that are closer to the Sun swing in their orbit to the opposite side of the Sun and appear to move in a different direction for a time. If you believe the Earth is the center of the Solar System, such motion is strange and puzzling and famously needed to be explained using a complicated system of epicycles which allowed a planet to move backwards at certain points in its motion. With the heliocentric Solar System, the explanation becomes as simple as explaining relative motion.
Our advancing understanding of the motions of the celestial sphere also mean that the claims of many so-called “classical” astrologers are based on calculations that place the planets in different places in the sky than they actually are. The Earth’s axis precesses every 26,000 years which means that theCelestial Poles point in slightly different directions with respect to the background stars year by year. This motion was unknown to ancient astronomers because it requires either a significant baseline of observations before the shifts are seeable without advanced technology. But it has been long enough since the invention of astrology that most people’s “Star Signs” are no longer the constellation that the Sun was in when they were born.
Finally, there is a question of mechanism when it comes to astrology. Some astrologers were probably encouraged by the fact that Isaac Newton’s explanation for gravity required the planets to exert gravitational pulls on human beings. However, the force exerted is minuscule compared to other forces we encounter in our day-to-day lives. The planet Jupiter exerts less of a gravitational influence on you than the gravity from a nearby human being; it is essentially too small to measure. As for the other forces known to physics (electromagnetism, the strong force, and the weak force), their influences are even smaller.
Thus, in order for astrology to work there would have to be some unknown fifth force originating from the planets, influencing humanity, and following the traditional stories that were told to explain motion of the planets that we now explain through celestial mechanics. Such a force should be measurable, but it does not appear in any of the tests that physicists make to look for such fifth forces. The conclusion that most physicists arrive at, then, is that astrological claims cannot attributable to actual physical influences.
At this point, some believers in astrology appeal to spirituality to argue that the influences cannot be measured. There are profound philosophical implications to believing in the existence of things that cannot be measured, well beyond the scope of this text. By definition, there is no test that can be done to either substantiate or debunk the existence of immeasurable things. We have left the purview of science and have entered into a space of ideas that is more properly contained within metaphysics or religion.
Spirituality and Religion
Some religions make claims directly relevant to the questions this book is seeking to explore. For example, there are those who have a religious belief that there cannot possibly be intelligent life in the Universe other than humanity. Others not only believe in such life, but make claims to know what the life is and how it operates. Some modern religions even incorporate UFOs as part of their belief systems.
Other religious believers question fundamental results of scientific investigation. However, most mainstream religions do not see any necessary conflict between scientific investigations, the facts discovered by science, and the religious beliefs. There may be outstanding queries and disagreements as to what is possible or impossible in such discussions of religion and science, but generally there is an ongoing demarcation that is maintained between religion and science that is only breached in certain philosophical fora. Students are often intrigued by this (lack of) interaction, but because the approaches to explaining the world are very different between a religious perspective and a scientific perspective, it is typically difficult to isolate even what the topics up for discussion should be. Open questions in science are sometimes left to religions to answer. Whether they provide reasonable answers or not is largely a question of faith.
Key Concepts and Summary
Without a second example of life elsewhere, the field of astrobiology is speculative. This offers an excellent opportunity to develop critical thinking, skepticism, and logic as thinking habits. It is human nature to want to succeed. It is scientific nature to be skeptical and demand empirical evidence before jumping to conclusions and the scientists working on SETI and the search for life employ these best practices in their work. They will be the first to say there is no evidence that UFOs are alien spacecraft or to identify hoaxes met to deceive. These practices may be the most important thing you can take away from your study of astrobiology.
Review Questions
Summary Questions
- Which item in Carl Sagan’s baloney detection kit do you think is most useful for identifying baloney? Give an example where it would be helpful in identifying a questionable claim.
- Are there ever instances where the baloney detection kit does not work well?
- Give an example of a false positive, that is, an instance where the baloney detection kit might indicate that there is something problematic about a scientific proposition when there is not anything problematic about it.
- Give an example of a false negative, that is, an instance where the baloney detection kit might not indicate that an idea is problematic when it is, in fact, pseudoscientific.
- Investigate a UFO claim and look for possible explanations that would involve natural phenomena, human technology, delusions, or hoaxes. Explain why a skeptic might find such an explanation more convincing than an explanation that it was caused by life from beyond the Earth.
- How might the belief that UFOs are caused by intelligent, non-human forms of life relate to some religious and/or spiritual beliefs?
Activities
- One of the best known cases of supposed alien artifacts is the recovery of the crash remnants of a “flying disk” in Roswell, New Mexico: the so-called Roswell incident of 1947. This episode would have been filed away with the myriad other flimsy allegations of alien evidence if not for the 1978 revival of the case, which pushed the incident from a claim to a conspiracy. Explore the seventy-year history of the Roswell incident and then present the case through a medium of your choice. Express the sequence of events in a painting, a poem, a skit, a comic, etc. presented to your class in an Alien Exhibition. Your piece must incorporate in some way the major episodes of the Roswell incident:
★ The 1947 discovery of the alien artifacts
★ The 1978 re-investigation of the case
★ The current state of the conspiracy
Your piece should also make a comment on the believability of the conspiracy according to what you have learned in your studies of astrobiology. Presentations should take no longer than 5 minutes for visual pieces and no longer than 10 minutes for reenactments (which can be pre-recorded and submitted electronically). Good luck!
👽 DO YOU WANT TO BELIEVE? 👽
the process by which scientific and other academic work is vetted and verified by other experts
an idea that seems scientific which, on closer scrutiny, turns out to be not based on scientific reasoning.
a philosophy that questions whether claims are made with strong or reasonable arguments and rejects those which are based on weak or unreasonable ones.
the claim in scientific research that the effect being studied does not exist
(1934-1996) astronomer and science communicator who worked on solar system science, the search for extraterrestrial intelligence, and skepticism
the adage that when faced with multiple explanations for a phenomenon, the simplest one is usually the best
Catch-all term used to describe claims of unusual, mysterious, and unexplainable sightings in the sky. Often associated with claims of extraterrestrial visitations.
Science fiction writer of the late nineteenth and early twentieth century.
Astronomer and wealthy entrepreneur who founded an observatory with the express interest of studying Mars and the now known-to-be erroneous claim that is had a network of canals on the surface.
Director of the Milan Observatory in the late nineteenth century.
sightings of airships, in the last half of the nineteenth century around the time of the invention of blimps or dirigibles, in areas where no airships were known to be flying