Chapter 9: Learning and Memory
9.5: Molecular Mechanisms of Learning
Zooming in beyond the level of anatomy, the substrates of learning can be found at the level of synapses. Synapses change in a phenomenon called plasticity. The word “plasticity” refers to a change in synaptic strength, which may be an increase or a decrease. This change may persist for minutes, hours, days, or in some cases, even a whole lifetime. When synaptic strength is increased and remains elevated, we call this long- term potentiation (LTP). A prolonged weakness of a synapse is called long-term depression (LTD). In our current limited understanding of plasticity, both phenomena are important for a healthy brain, and neither one is always good or always bad.
It is also important to clarify that both excitatory synapses and inhibitory synapses can be subject to either LTP or LTD.
Long-term potentiation
In 1973, Bliss and Lomo were the first to publish evidence of plasticity using electrophysiology. The experiment began at the hippocampal connections of an anesthetized rabbit. They put a stimulating electrode among the axons of the perforant pathway, at the entrance to the hippocampus. A second electrode, capable of detecting electrical charges in brain tissue, was placed among the cells of the dentate gyrus, the area where those axons release neurotransmitter. By stimulating the perforant pathway, Bliss and Lomo could record how neurons of the dentate gyrus respond. A single pulse caused the neurons to depolarize, a measurable observation called a field excitatory post-synaptic potential (fEPSP). The more neurons that depolarize, the larger the fEPSP would be.
Instead of simply giving a single electrical stimulation, however, Bliss and Lomo were interested in testing Hebb’s theory about plasticity. If “cells that fire together, wire together,” then perhaps they could experimentally drive those cells to fire in a pattern that would induce a rewiring of the connections, resulting in LTP. The duo delivered a very intense electrical stimulation, zapping the axons at 100 stimulations a second (100 Hz) for 3 seconds. This high frequency stimulation (HFS) led to an enhancement of the amplitude of the fEPSP in response to a single stimulus – this demonstrated that LTP was a measurable phenomenon. In a different experimental setup, this LTP was shown to persist up to one year later! In humans, we theorize that some synaptic connections may remain potentiated for our entire lifetime, however investigating this is in humans is ethically constrained.
Long-lasting changes in synaptic strength, such as the LTP that Bliss and Lomo demonstrated, are made possible through a series of molecular and cellular level changes. One form of LTP results from a change in the types of glutamatergic receptors. Of the three classes of ionotropic glutamate receptors, two are important for this form of LTP: the AMPA and the NMDA receptors. The AMPA receptors are the glutamate receptors that we generally imagine as contributing to excitation (more information in section 5.4). When a molecule of glutamate binds to the active site of this receptor, the ligand-gated ion channel changes and allows cations, mostly Na+, to cross the cell membrane, leading to depolarization.

The NMDA receptors are somewhat more complex. NMDA receptors are like the ionotropic AMPA receptors because they are permeable to cations and therefore excitatory, but they have a few specific functional differences. For one, their molecular pore has space for a magnesium ion (Mg2+) to sit in the middle of the ion channel. Mg2+, like the other ions that we have discussed (chapter 4.2), responds similarly to the forces of the electrochemical gradient. Mg2+ is more highly concentrated outside the cell compared to the inside, and it has two positive charges, so these ions are drawn to the interior of the cell. But, the pore of the NMDA receptor is not large enough to allow the bulky Mg2+ ion to actually cross into or out of the cell membrane. Instead, it stays stuck inside the ion channel. Mg2+ physically takes up so much space that it occludes the movement of other ions across the cell membrane, basically blocking passage of ions through the NMDA receptor.
The other relevant feature of the NMDA receptor is that it is permeable to the Ca2+ ion. Increases in intracellular Ca2+ postsynaptically is the crucial trigger that leads to the cellular expression of LTP. Ca2+ ions activate an enzyme called calcium / calmodulin-dependent protein kinase II (CaMKII). CaMKII itself has many molecular targets. As a kinase, it’s main molecular action is to phosphorylate proteins. When CaMKII becomes activated, it phosphorylates amino acid residues on the AMPA receptor, which enhances their current passing properties, thereby increasing their response with glutamate present. Secondly, CaMKII also contributes to cellular mechanisms which result in increased trafficking of AMPA receptors to the cell surface. Thirdly, CaMKII interacts with the transcription factor cAMP response element-binding protein (CREB), which can then move into the nucleus and instruct the nucleus to synthesize more of the mRNA that leads to increased synthesis of the AMPA receptors. Taken together, increases in intracellular Ca2+ postsynaptically leads to an enhancement of a signal that persists over the time course of hours: The definition of LTP.
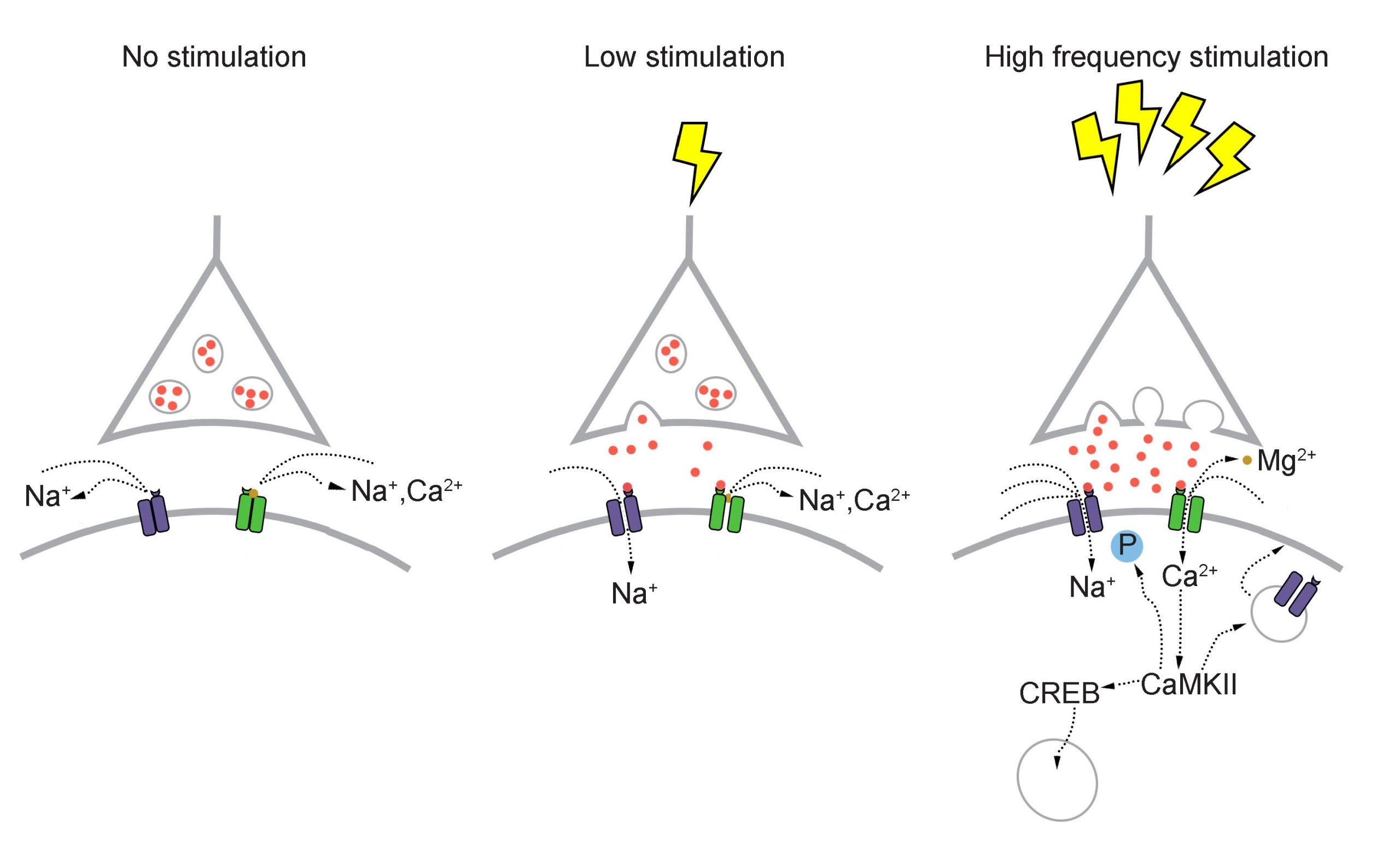
But, these NMDA receptors are not activated by glutamate alone. Because of themolecular properties of the NMDA receptors, they need two conditions to be fulfilled before these receptors get activated and Ca2+ moves into the cell membrane:
- A ligand like glutamate must activate the receptor. As with other receptors, there is no activation in the absence of an agonist.
- The postsynaptic cell must also be depolarized. When the cell is at positive potentials, the electrical gradient causes the bulky Mg2+ ion that is stuck in the pore to be repelled by the cell’s interior, which then frees the ion channel for movement of cations across the cell membrane.
Because both conditions must be met before Ca2+ can trigger plasticity through CaMKII activation, the NMDA receptor can be described as a coincidence detector. These properties can help explain why LTP was only observed after Bliss and Lomo activated the hippocampal slices robustly with their high frequency stimulus paradigm. Strong activation depolarized the axonal fibers, which caused a significant amount of glutamate to be released, activating many of the postsynaptic AMPA receptors. This strong activation caused the postsynaptic neurons to depolarize, which expels the Mg2+ ion out of the NMDA receptor. At this stage, both conditions are fulfilled, and Ca2+ enters into the postsynaptic cell, which activates CaMKII, triggering LTP.
Some glutamatergic connections between neurons contain only NMDA receptors but no AMPA receptors. Because these postsynaptic cells do not depolarize in response to glutamate release, and no current passes through the NMDA receptor due to the Mg2+ block, these synapses do not change their activity even with glutamate release. These synapses are called silent synapses. As we grow, the number of silent synapses decreases, another aspect of brain development.
Long-term depression
Around the same tine that LTP was being characterized in the rabbit hippocampus, its cellular opposite, long-term depression (LTD), was also being demonstrated in a different experimental preparation. Through the 60s, psychiatrist Eric Kandel and his colleagues worked with the marine mollusk Aplysia californica. With a nervous system of only 20,000 cells, Aplysia is orders of magnitude simpler than the other model organisms used at the time. Additionally, some Aplysia neurons are huge, up to a millimeter in diameter, which took away the need for highly precise equipment.
Aplysia also has a relatively simple anatomy. It breathes using a half-circle of delicate tissue called the gill, which is guarded by the mantle shelf. They also have an organ called the siphon, a small tube that is used for moving water through the animal. Kandel and his colleagues began their exploration of memory by studying the gill-withdrawal reflex, a defensive motor response behavior. When a stimulus, such as a hungry predator (or an experimenter’s paintbrush), grazed the siphon, the Aplysia would reflexively withdraw their gill, as if to protect this vital organ by shrinking away from the threat. However, after repeated brush strokes to the siphon, the sea slugs figured out that the stimulus was completely innocuous, and decreased the strength of gill withdrawal. Kandel and his team suggested that this change in behavior (habituation) was a form of learning.
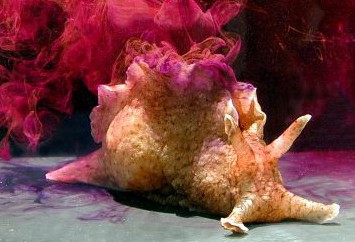
Kandel discovered evidence of habituation, the suppression of a normal reflex behavior that is dependent on LTD. To further explore the cellular and molecular level changes behind this LTD, they conducted electrophysiological experiments on Aplysia. The gill-withdrawal reflex circuit relies heavily on two different populations of neurons: the sensory neurons that receive somatosensory information from the skin of the siphon, and the motor neurons that control the muscles of the gill. By using two different tiny glass pipettes, they could impale these neurons, inducing action potential firing in the sensory neuron, and observe changes in membrane potential of the motor neurons (a depolarization of the membrane of a single neuron is called an excitatory post- synaptic potential, or EPSP). When the sensory neuron was activated, they observed an EPSP in response, since an action potential caused release of glutamate that activates post-synaptic receptors on the motor neuron. However, after the reflex had been habituated, the same sensory neuron activation caused a much smaller EPSP in the motor neuron.
The group went about seeing if they could modify this habituated response, curious if a stored memory can be modified by stimuli from the outside world. When they paired the mild siphon touch with a painful electric shock to the tail, the Aplysia began responding with a strong motor reaction, withdrawing the gill very intensely, indicating that the inhibited response disappeared. They called this observation sensitization. In electrophysiological studies, they observed that the EPSP at the motor neuron was much larger following the tail shock.
On a molecular level, presentation of sensitization is downstream of the action of a third population of neurons, interneurons that synapse onto the motor neurons. The noxious stimulus triggers these interneurons to release the neurotransmitter serotonin, which activates the excitatory signaling molecule cAMP found at the terminals of the motor neurons, thus increasing release probability and strengthening the gill withdrawal reflex.
