3 Conception and Heredity
Learning Objectives
After Reading Chapter 3, you should be better equipped to:
- Evaluate the role of nature and nurture in development.
- Define genes and chromosomes.
- Differentiate between mitosis and meiosis.
- Explain dominant and recessive patterns on inheritance.
- List common genetic disorders and chromosomal abnormalities.
- Describe changes that occur within each of the three periods of prenatal development.
- Recognize the risks to prenatal development posed by exposure to teratogens.
- Evaluate different types of prenatal assessment.
Heredity
The Human Genome Project
The Human Genome Project is an internationally funded effort to map the locations of human genes and understand the role these genes play in development, health, and illness. (recent developments can be found at www.genome.gov). Genes are segments of chromosomes (46 strands of a chemical substance called DNA that are contained in the nucleus of each normal human cell) that vary in length. There are an estimated 25,000 to 30,000 genes on each chromosome; a number far below the estimate of 100,000-150,000 held before the work of the Human Genome Project.
Illustration of DNA’s Location in a Cell [2]
Illustration of location of DNA on a chromosome. Chromosomes are found in the nucleus of a cell.
Understanding the role of genes in health and illness can bring about both harm and good (Weitz, 2007). A person who knows that they are at risk for developing a genetic disorder may be able to adopt lifestyle practices that minimize the risk and a person who discovers that they are not at risk may find comfort in knowing that they do not have to fear a particular disease. However, a person who finds out that they are at risk and there is nothing that can be done about it may experience years of fear and anxiety. And the availability of genetic testing may be more widespread than the availability of genetic counseling which can be very expensive. The possible stigma and discrimination that those with illness or at risk for illness must also be considered. Considering the high costs of health insurance, many companies are starting to offer benefits contingent on health assessments and lifestyle recommendations; and continued coverage depends on an employee following these recommendations. So, a smoker may have to pay a higher premium than a non-smoker or a person who is overweight may be required to engage in a program of exercise and be monitored for improvement. What if a person finds out that they carry the gene for Huntington’s disease (a neurological disorder that is ultimately fatal) which may surface when a person reaches their 40s? The impact this knowledge will have on health care continues to remain unknown. Who should know what is on your genome? Do you think this information should be shared between mates? What about employers? What would be the advantages and disadvantages?[1]
Chromosomes: Mitosis and Meiosis
Normal human cells contain 46 chromosomes (or 23 pairs; one from each parent) in the nucleus of the cells. After conception, most cells of the body are created by a process of cell duplication called mitosis. Strictly applied, the term mitosis is used to describe the duplication and distribution of chromosomes, the structures that carry the genetic information. Prior to the onset of mitosis, the chromosomes have replicated and the proteins that will form the mitotic spindle have been synthesized. Mitosis begins at prophase with the thickening and coiling of the chromosomes. The nucleolus, a rounded structure, shrinks and disappears. The end of prophase is marked by the beginning of the organization of a group of fibres to form a spindle and the disintegration of the nuclear membrane.
The chromosomes, each of which is a double structure consisting of duplicate chromatids, line up along the midline of the cell at metaphase. In anaphase each chromatid pair separates into two identical chromosomes that are pulled to opposite ends of the cell by the spindle fibers. During telophase, the chromosomes begin to decondense, the spindle breaks down, and the nuclear membranes and nucleoli re-form. The cytoplasm of the mother cell divides to form two daughter cells, each containing the same number and kind of chromosomes as the mother cell. The stage, or phase, after the completion of mitosis is called interphase.
Mitosis is absolutely essential to life because it provides new cells for growth and for replacement of worn-out cells. Mitosis may take minutes or hours, depending upon the kind of cells and species of organisms. It is influenced by time of day, temperature, and chemicals.[2]
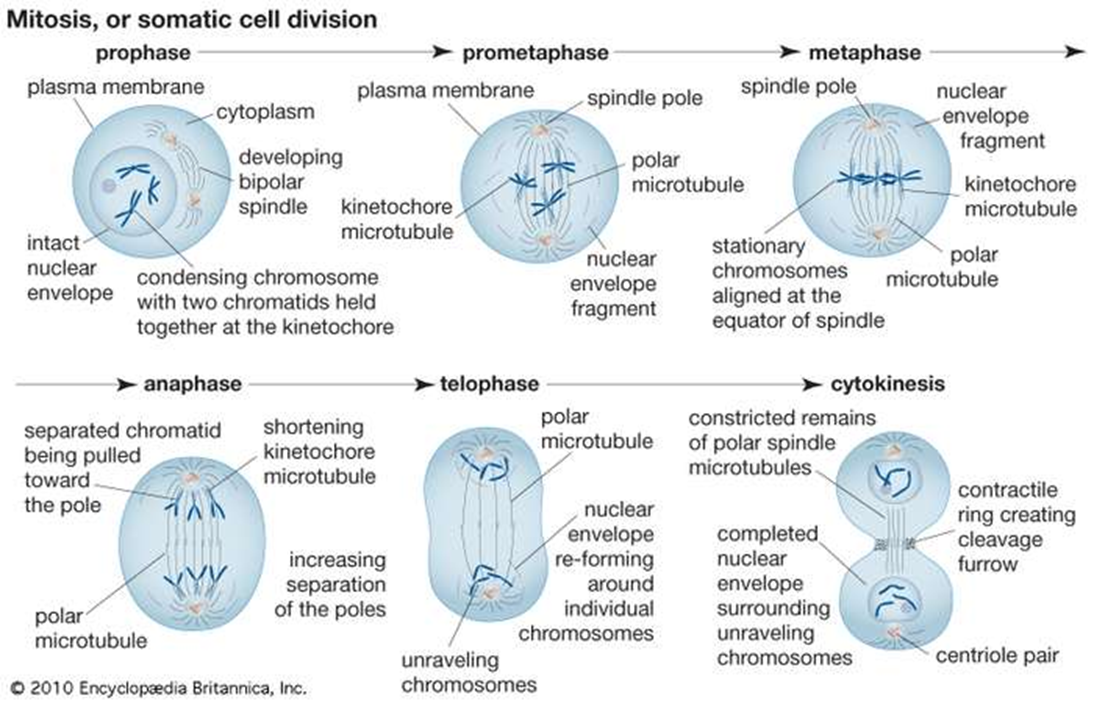
However, the cells used in sexual reproduction, called gametes (sperm or ova), are formed in a process called meiosis. Meiosis is the nuclear division that forms haploid cells from diploid cells, and it employs many of the same cellular mechanisms as mitosis. However, as you have learned, mitosis produces daughter cells whose nuclei are genetically identical to the original parent nucleus. In meiosis, the starting nucleus is always diploid and the daughter nuclei that result are haploid. Thus, each sperm and egg possess only 23 chromosomes and combine to produce the normal 46. To achieve this reduction in chromosome number, meiosis consists of one round of chromosome replication followed by two rounds of nuclear division. Because many events that occur during each of the division stages are analogous to the events of mitosis, the same stage names are assigned. However, because there are two rounds of division, the major process and the stages are designated with a “I” or a “II.” Thus, meiosis I is the first round of meiotic division and consists of prophase I, prometaphase I, and so on. Likewise, Meiosis II (during which the second round of meiotic division takes place) includes prophase II, prometaphase II, and so on. Given the number of genes present and the unpredictability of the meiosis process, the likelihood of having offspring that are genetically identical (and not twins) is one in trillions (Gould & Keeton, 1997).[3]
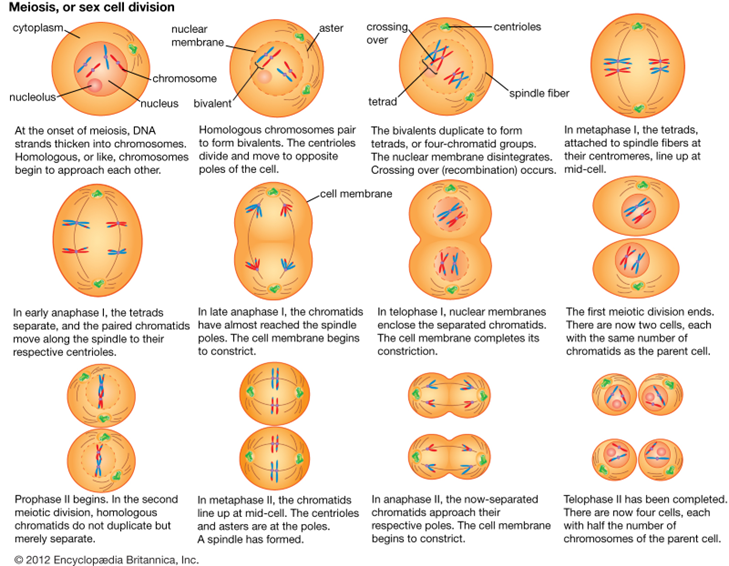
Summary of Mitosis and Meiosis[4]
From Genotype to Phenotype
Each human body cell has a full complement of DNA stored in 23 pairs of chromosomes. The image below shows the pairs in a systematic arrangement called a karyotype. Among these is one pair of chromosomes, called the sex chromosomes, that determines the sex of the individual (XX in females, XY in males). The remaining 22 chromosome pairs are called autosomal chromosomes or autosomes. Each of these chromosomes carries hundreds or even thousands of genes, each of which codes for the assembly of a particular protein—that is, genes are “expressed” as proteins. An individual’s complete genetic makeup is referred to as his or her genotype. The characteristics that the genes express, whether they are physical, behavioral, or biochemical, are a person’s phenotype.
You inherit one chromosome in each pair—a full complement of 23—from each parent. This occurs when the sperm and oocyte combine at the moment of your conception. Homologous chromosomes—those that make up a complementary pair—have genes for the same characteristics in the same location on the chromosome. Because one copy of a gene, is inherited from each parent, the alleles in these complementary pairs may vary. Take for example an allele that encodes for dimples. A child may inherit the allele encoding for dimples on the chromosome from the father and the allele that encodes for smooth skin (no dimples) on the chromosome from the mother. The following figure shows the 23 pairs of chromosomes in both a male and female human being.[5]
Examples of Healthy Male and Female Karyotypes
Each pair of chromosomes contains hundreds to thousands of genes. The banding patterns are nearly identical for the two chromosomes within each pair, indicating the same organization of genes. As is visible in this karyotype, the only exception to this is the XY sex chromosome pair in males shown on the left. The karyotype on the right shows the XX sex chromosomes of females. (credit: National Human Genome Research Institute). [6]
Although a person can have two identical alleles for a single gene (a homozygous state), it is also possible for a person to have two different alleles (a heterozygous state). The two alleles can interact in several different ways. The expression of an allele can be dominant, for which the activity of this gene will mask the expression of a nondominant, or recessive, allele. Sometimes dominance is complete; at other times, it is incomplete. In some cases, both alleles are expressed at the same time in a form of expression known as codominance.
In the simplest scenario, a single pair of genes will determine a single heritable characteristic. However, it is quite common for multiple genes to interact to confer a feature. For instance, eight or more genes—each with their own alleles—determine eye color in humans. Moreover, although any one person can only have two alleles corresponding to a given gene, more than two alleles commonly exist in a population. This phenomenon is called multiple alleles. For example, there are three different alleles that encode ABO blood type.[7]
Mendel’s Theory of Inheritance
Our contemporary understanding of genetics rests on the work of a nineteenth-century monk. Working in the mid-1800s, long before anyone knew about genes or chromosomes, Gregor Mendel discovered that garden peas transmit their physical characteristics to subsequent generations in a discrete and predictable fashion. When he mated, or crossed, two pure-breeding pea plants that differed by a certain characteristic, the first-generation offspring all looked like one of the parents. For instance, when he crossed tall and dwarf pure-breeding pea plants, all of the offspring were tall. Mendel called tallness dominant because it was expressed in offspring when it was present in a purebred parent. He called dwarfism recessive because it was masked in the offspring if one of the purebred parents possessed the dominant characteristic. Note that tallness and dwarfism are variations on the characteristic of height. Mendel called such a variation a trait. We now know that these traits are the expression of different alleles of the gene encoding height.
Mendel performed thousands of crosses in pea plants with differing traits for a variety of characteristics. And he repeatedly came up with the same results—among the traits he studied, one was always dominant, and the other was always recessive. (Remember, however, that this dominant–recessive relationship between alleles is not always the case; some alleles are codominant, and sometimes dominance is incomplete.)
In the language of genetics, Mendel’s theory applied to humans says that if an individual receives two dominant alleles, one from each parent, the individual’s phenotype will express the dominant trait. If an individual receives two recessive alleles, then the recessive trait will be expressed in the phenotype. Individuals who have two identical alleles for a given gene, whether dominant or recessive, are said to be homozygous for that gene (homo- = “same”). Conversely, an individual who has one dominant allele and one recessive allele is said to be heterozygous for that gene (hetero- = “different” or “other”). In this case, the dominant trait will be expressed, and the individual will be phenotypically identical to an individual who possesses two dominant alleles for the trait.
It is common practice in genetics to use capital and lowercase letters to represent dominant and recessive alleles. Using Mendel’s pea plants as an example, if a tall pea plant is homozygous, it will possess two tall alleles (TT). A dwarf pea plant must be homozygous because its dwarfism can only be expressed when two recessive alleles are present (tt). A heterozygous pea plant (Tt) would be tall and phenotypically indistinguishable from a tall homozygous pea plant because of the dominant tall allele.
Because of the random segregation of gametes, the laws of chance and probability come into play when predicting the likelihood of a given phenotype. Consider a cross between an individual with two dominant alleles for a trait (AA) and an individual with two recessive alleles for the same trait (aa), as is shown in the Punnett Square below. All of the parental gametes from the dominant individual would be A, and all of the parental gametes from the recessive individual would be a. All of the offspring of that second generation, inheriting one allele from each parent, would have the genotype Aa, and the probability of expressing the phenotype of the dominant allele would be 4 out of 4, or 100 percent as is illustrated in the Punnett Square (a Punnett square is a diagram used to predict possible genotypes) below.[8]
Punnett Square Probable Outcomes
Possible outcomes of cross between one individual with homozygous, dominant alleles and one individual with homozygous recessive alleles[9]
Test Yourself :
Mendelian genetics represent the fundamentals of inheritance, but there are two important qualifiers to consider when applying Mendel’s findings to inheritance studies in humans. First, as we’ve already noted, not all genes are inherited in a dominant–recessive pattern. Although all diploid individuals have two alleles for every gene, allele pairs may interact to create several types of inheritance patterns, including incomplete dominance and codominance.
Secondly, Mendel performed his studies using thousands of pea plants and this large sample size overcame the influence of variability resulting from chance. In contrast, no human couple has ever had thousands of children. If we know that a man and woman are both heterozygous for a recessive genetic disorder, we would predict that one in every four of their children would be affected by the disease. In real life, however, the influence of chance could change that ratio significantly. For example, if a man and a woman are both heterozygous for cystic fibrosis, a recessive genetic disorder that is expressed only when the individual has two defective alleles, we would expect one in four of their children to have cystic fibrosis. However, it is entirely possible for them to have seven children, none of whom is affected, or for them to have two children, both of whom are affected. For each individual child, the presence or absence of a single gene disorder depends on which alleles that child inherits from his or her parents.[10]
Autosomal Dominant Inheritance
In the case of cystic fibrosis, the disorder is recessive to the normal phenotype. However, a genetic abnormality may be dominant to the normal phenotype. When the dominant allele is located on one of the 22 pairs of autosomes (non-sex chromosomes), we refer to its inheritance pattern as autosomal dominant. An example of an autosomal dominant disorder is neurofibromatosis type I, a disease that induces tumor formation within the nervous system that leads to skin and skeletal deformities. Consider a couple in which one parent is heterozygous for this disorder (and who therefore has neurofibromatosis), Nn, and one parent is homozygous for the normal gene, nn. The heterozygous parent would have a 50 percent chance of passing the dominant allele for this disorder to his or her offspring, and the homozygous parent would always pass the normal allele. Therefore, four possible offspring genotypes are equally likely to occur: Nn, Nn, nn, and nn. That is, every child of this couple would have a 50 percent chance of inheriting neurofibromatosis.[11]
Possible Outcomes When One Parent Carries an Autosomal Dominant Trait
Punnett Square showing the possible outcomes between one individual who is heterozygous for neurofibromatosis (Nn) and one who is homozygous and does not carry the dominant trait disorder of neurofibromatosis (nn). In order to develop and autosomal dominant disord the individual only needs to inherit the gene change from one parent.[12]
Other genetic diseases that are inherited in this pattern are achondroplastic dwarfism, Marfan syndrome, and Huntington’s disease. Because autosomal dominant disorders are expressed by the presence of just one gene, an individual with the disorder will know that he or she has at least one faulty gene. The expression of the disease may manifest later in life, after the childbearing years, which is the case in Huntington’s disease.[13]
Examples of Autosomal Dominant Disorders (Heterozygous) [14]
Disorder | Description | Cases per Birth |
Huntington’s Disease | A condition that affects the individual’s nervous system. Nerve cells become damaged, causing various parts of the brain to deteriorate. The disease affects movement, behavior and cognition. It is fatal, and occurs at midlife. | 1 in 10,000 |
Tourette Syndrome | A tic disorder which results in uncontrollable motor and vocal tics as well as body jerking | 1 in 250 |
Achondroplasia | The most common form of disproportionate short stature. The individual has abnormal bone growth resulting in short stature, disproportionately short arms and legs, short fingers, a large head, and specific facial features. | 1 in 15,000-40,000 |
Test Yourself:
Autosomal Recessive Inheritance
When a genetic disorder is inherited in an autosomal recessive pattern, the disorder corresponds to the recessive phenotype. Heterozygous individuals will not display symptoms of this disorder, because their unaffected gene will compensate. Such an individual is called a carrier. Carriers for an autosomal recessive disorder may never know their genotype unless they have a child with the disorder.
An example of an autosomal recessive disorder is cystic fibrosis (CF), which we introduced earlier. CF is characterized by the chronic accumulation of a thick, tenacious mucus in the lungs and digestive tract. Decades ago, children with CF rarely lived to adulthood. With advances in medical technology, the average lifespan in developed countries has increased into middle adulthood. CF is a relatively common disorder that occurs in approximately 1 in 2000 Caucasians. A child born to two CF carriers would have a 25 percent chance of inheriting the disease. The pattern is shown in the image below, using a diagram that tracks the likely incidence of an autosomal recessive disorder on the basis of parental genotypes.[15]
On the other hand, a child born to a CF carrier and someone with two unaffected alleles would have a 0 percent probability of inheriting CF but would have a 50 percent chance of being a carrier. Other examples of autosome recessive genetic illnesses include the blood disorder sickle-cell anemia, the fatal neurological disorder Tay–Sachs disease, and the metabolic disorder phenylketonuria.[16]
Probabilities of Autosomal Recessive Inheritance
The inheritance pattern of an autosomal recessive disorder with two carrier parents reflects a 3:1 probability of expression among offspring. (credit: U.S. National Library of Medicine) [17] If the gene is inherited from just one parent, the person is a carrier and does not have the condition. Recessive gene disorders, such as cystic fibrosis and sickel-cell anemia, are less common but may claim more lives because they are less likely to be detected.[18]
Examples of Recessive Trait Disorders (Homozygous)[19]
Disorder | Description | Cases per Birth |
Sickle Cell Disease (SCD) | A condition in which the red blood cells in the body are shaped like a sickle (like the letter C) and affect the ability of the blood to transport oxygen. | 1 in 500 Black births
1 in 36,000 Hispanic births |
Cystic Fibrosis (CF) | A condition that affects breathing and digestion due to thick mucus building up in the body, especially the lungs and digestive system. In CF, the mucus is thicker than normal and sticky. | 1 in 3500 |
Phenylketonuria (PKU) | A metabolic disorder in which the individual cannot metabolize phenylalanine, an amino acid. Left untreated, intellectual deficits occur. PKU is easily detected and is treated with a special diet. | 1 in 10,000 |
Tay Sachs Disease | Caused by an enzyme deficiency resulting in the accumulation of lipids in the nerves cells of the brain. This accumulation results in progressive damage to the cells and a decrease in cognitive and physical development. Death typically occurs by age five. | 1 in 4000
1 in 30 American Jews is a carrier 1 in 20 French Canadians is a carrier |
Albinism | When the individual lacks melanin and processes little to no pigment in the skin, hair, and eyes. Vision problems can also occur. | Fewer than 20,000 US cases per year |
Test Yourself:
Sex-Linked Disorders
When the X chromosome carries the mutated gene, the disorder is referred to as an X-linked disorder. Males are more affected than females because they possess only one X chromosome without an additional X chromosome to counter the harmful gene.[20]
X-linked Dominant and Recessive Inheritance
An X-linked transmission pattern involves genes located on the X chromosome of the 23rd pair. Recall that a male has one X and one Y chromosome. When a father transmits a Y chromosome, the child is male, and when he transmits an X chromosome, the child is female. A mother can transmit only an X chromosome, as both her sex chromosomes are X chromosomes.
X-linked Dominant Inheritance
When an abnormal allele for a gene that occurs on the X chromosome is dominant over the normal allele, the pattern is described as X-linked dominant. This is the case with vitamin D–resistant rickets: an affected father would pass the disease gene to all of his daughters, but none of his sons, because he donates only the Y chromosome to his sons. If it is the mother who is affected, all of her children—male or female—would have a 50 percent chance of inheriting the disorder because she can only pass an X chromosome on to her children. For an affected female, the inheritance pattern would be identical to that of an autosomal dominant inheritance pattern in which one parent is heterozygous and the other is homozygous for the normal gene.[21]
Probabilities of X-Linked Dominant Inheritance
Chart depicting X-linked dominant inheritance patterns and how that pattern changes depending on whether (a) the father or (b) the mother is affected with the disease. (Credit: U.S. National Library of Medicine).
Test Yourself:
X-linked Recessive Inheritance
X-linked recessive inheritance refers to genetic conditions associated with mutations in genes on the X chromosome. A male carrying such a mutation will be affected, because he carries only one X chromosome. A female carrying a mutation in one gene, with a normal gene on the other X chromosome, is generally unaffected.[22][23]
Probabilities of X-Linked Dominant Inheritance
X-linked recessive inheritance is a way a genetic trait or condition can be passed down from parent to child through mutations (changes) in a gene on the X chromosome. In males (who only have one X chromosome), a mutation in the copy of the gene on the single X chromosome causes the condition. Females (who have two X chromosomes) must have a mutation on both X chromosomes in order to be affected with the condition. If only the father or the mother has the mutated X-linked gene, the daughters are usually not affected and are called carriers because one of their X chromosomes has the mutation but the other one is normal. Sons will be affected if they inherit the mutated X-linked gene from their mother. Fathers cannot pass X-linked recessive conditions to their sons.
Test Yourself:
Other Inheritance Patterns
Incomplete Dominance
Mendel’s results, that traits are inherited as dominant and recessive pairs, contradicted the view at that time that offspring exhibited a blend of their parents’ traits. However, the heterozygote phenotype occasionally does appear to be intermediate between the two parents. For example, in the snapdragon, Antirrhinum majus (Figure below), a cross between a homozygous parent with white flowers (CWCW) and a homozygous parent with red flowers (CRCR) will produce offspring with pink flowers (CRCW). (Note that different genotypic abbreviations are used for Mendelian extensions to distinguish these patterns from simple dominance and .) This pattern of inheritance is described as incomplete dominance, denoting the expression of two contrasting alleles such that the individual displays an intermediate phenotype. The allele for red flowers is incompletely dominant over the allele for white flowers. However, the results of a heterozygote self-cross can still be predicted, just as with Mendelian dominant and recessive crosses. In this case, the genotypic ratio would be 1 CRCR:2 CRCW:1 CWCW, and the phenotypic ratio would be 1:2:1 for red:pink:white.
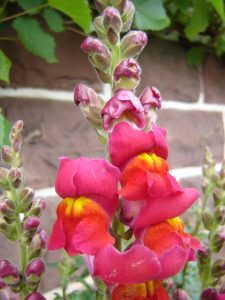
Incomplete dominance can be seen in several types of flowers, including pink tulips, carnations and roses—any pink flowers in these are due to the mixing of red and white alleles. Incomplete dominance can also be observed in some animals, such as rabbits. When a long-furred Angora breeds with a short-furred Rex, the offspring have medium-length fur. Tail length in dogs is similarly impacted by genes that display incomplete dominance patterns. For humans wavy hair, and voice pitch can be affected by incomplete dominance patterns.[24]
Codominance
A variation on incomplete dominance is codominance, in which both alleles for the same characteristic are simultaneously expressed in the heterozygote. An example of codominance is the MN blood groups of humans. The M and N alleles are expressed in the form of an M or N antigen present on the surface of red blood cells. Homozygotes (LMLM and LNLN) express either the M or the N allele, and heterozygotes (LMLN) express both alleles equally. In a self-cross between heterozygotes expressing a codominant trait, the three possible offspring genotypes are phenotypically distinct. However, the 1:2:1 genotypic ratio characteristic of a Mendelian monohybrid cross still applies.
Codominance can also be seen in human blood types: the AB blood type is a result of both the IA allele and the IB allele being codominant. The roan coat color in horses is also an example of codominance. A “red” roan results from the mating of a chestnut parent and a white parent (Figure 2). We know this is codominance because individual hairs are either chestnut or they are white, leading to the red roan overall appearance (see image below).[25]
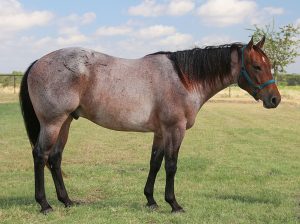
So what’s the difference between incomplete dominance and codominant inheritance? While they are very similar, the key difference is this: in incomplete dominance, the two traits are blended together, whereas in codominance, both traits are expressed.[26]
Lethal Alleles
The dominant lethal inheritance pattern is one in which an allele is lethal both in the homozygote and the heterozygote. Individuals with dominant lethal alleles fail to survive even in the heterozygote form. Dominant lethal alleles are very rare because, as you might expect, the allele only lasts one generation and is not transmitted.[27]
Lethal recessive alleles cause pre- or postnatal death in homozygous affected individuals, reducing fertility in various populations. Although recessive lethals are generally widespread throughout populations, their effect is generally masked since both alleles are necessary for the disease to occur. However, within small sized domestic and wild populations, those alleles might be exposed by inbreeding, caused by matings between related parents that inherited the same recessive lethal allele from a common ancestor. The precise impact of recessive lethals depends on the population structure (i.e. effective population size) and recessive lethal mutation rates. For example, in livestock, populations have been subject to intensive (genomic) selection resulting in relative small effective population sizes. With small effective population size, genetic drift can rapidly increase the frequency of recessive lethals in the population. [28]
Like the recessive lethal allele might not immediately manifest the phenotype of death, dominant lethal alleles also might not be expressed until adulthood. Once the individual reaches reproductive age, the allele may be unknowingly passed on, resulting in a delayed death in both generations. An example of this in humans is Huntington’s disease in which the nervous system gradually wastes away. People who are heterozygous for the dominant Huntington allele (Hh) will inevitably develop the fatal disease. However, the onset of Huntington’s disease may not occur until age 40, at which point the afflicted persons may have already passed the allele to 50 percent of their offspring.[29]
Genetic Mutations
Over a lifetime, our DNA can undergo changes or mutations in the sequence of bases: A, C, G and T. This results in changes in the proteins that are made, and this change can be a bad or a good thing.
A mutation is a change that occurs in our DNA sequence, either due to mistakes when the DNA is copied or as the result of environmental factors such as UV light and cigarette smoke. Mutations can occur during DNA replication if errors are made and not corrected in time. Mutations can also occur as the result of exposure to environmental factors such as smoking, sunlight and radiation. Often cells can recognize any potentially mutation-causing damage and repair it before it becomes a fixed mutation.
Mutations contribute to genetic variation within species. Mutations can also be inherited, particularly if they have a positive effect. For example, the disorder sickle cell anemia is caused by a mutation in the gene that instructs the building of a protein called hemoglobin. This causes the red blood cells to become an abnormal, rigid, sickle shape. However, in African populations, having this mutation also protects against malaria.
However, mutation can also disrupt normal gene activity and cause diseases, like cancer. Cancer is the most common human genetic disease; it is caused by mutations occurring in a number of growth-controlling genes. Sometimes faulty, cancer-causing genes can exist from birth, increasing a person’s chance of getting cancer.[30]
Chromosomal Disorders
A chromosomal abnormality occurs when a child inherits too many or too few chromosomes. Cases where a child inherits three chromosomes are referred to as trisomy’s and cases where a child only inherits one chromosome are called monosomy’s The most common cause of chromosomal abnormalities is the age of the mother. A 20-year-old woman has a 1 in 800 chance of having a child with a common chromosomal abnormality. A woman of 44, however, has a one in 16 chance. It is believed that the problem occurs when the ovum is ripening prior to ovulation each month. As the mother ages, the ovum is more likely to suffer abnormalities at this time.
Another common cause of chromosomal abnormalities occurs because the gametes do not divide evenly when they are forming. Therefore, some cells have more than 46 chromosomes. In fact, it is believed that close to half of all zygotes have an odd number of chromosomes. Most of these zygotes fail to develop and are spontaneously aborted by the body. If the abnormal number occurs on pair # 21 or # 23, however, the individual may have certain physical or other abnormalities.
One of the most common chromosomal abnormalities is on pair # 21. Trisomy 21 or Down Syndrome occurs when there are three rather than two chromosomes on #21. A person with Down syndrome has distinct facial features, intellectual development disorder (intellectual disability), and oftentimes heart and gastrointestinal disorders. Symptoms vary from person to person and can range from mild to severe. With early intervention, the life expectancy of persons with Down syndrome has increased in recent years. Keep in mind that there is as much variation in people with Down Syndrome as in most populations and those differences need to be recognized and appreciated.[31] [32]
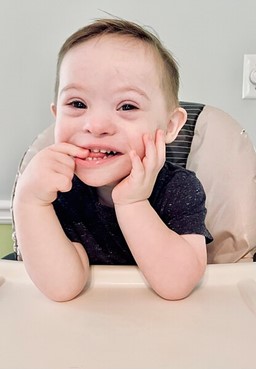
Trisomy 13, also called Patau syndrome, is a chromosomal condition associated with severe intellectual disability and physical abnormalities in many parts of the body. Individuals with trisomy 13 often have heart defects, brain or spinal cord abnormalities, very small or poorly developed eyes (microphthalmia), extra fingers or toes, an opening in the lip (a cleft lip) with or without an opening in the roof of the mouth (a cleft palate), and weak muscle tone (hypotonia). Due to the presence of several life-threatening medical problems, many infants with trisomy 13 die within their first days or weeks of life. Only five percent to 10 percent of children with this condition live past their first year.[33] [34]
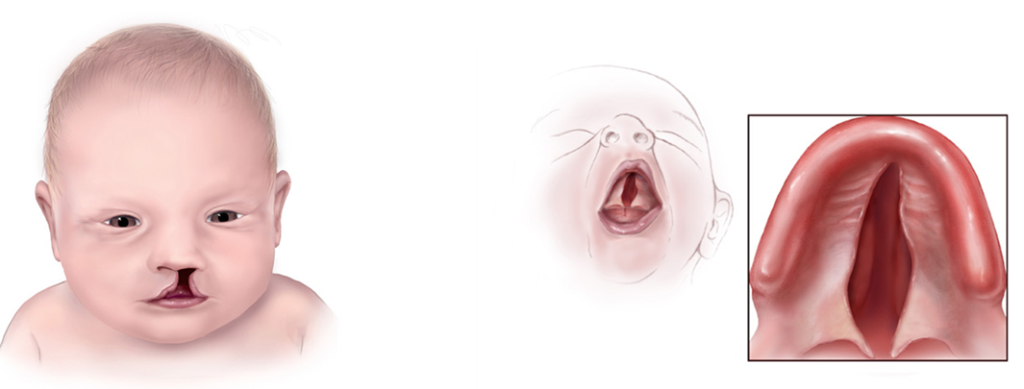
Trisomy 18, also called Edwards syndrome, is a chromosomal condition associated with abnormalities in many parts of the body. Individuals with trisomy 18 often have slow growth before birth (intrauterine growth retardation) and a low birth weight. Affected individuals may have heart defects and abnormalities of other organs that develop before birth. Other features of trisomy 18 include a small, abnormally shaped head; a small jaw and mouth; and clenched fists with overlapping fingers. Due to the presence of several life-threatening medical problems, many individuals with trisomy 18 die before birth or within their first month. Five to 10 percent of children with this condition live past their first year, and these children often have severe intellectual disability.[35] [36]
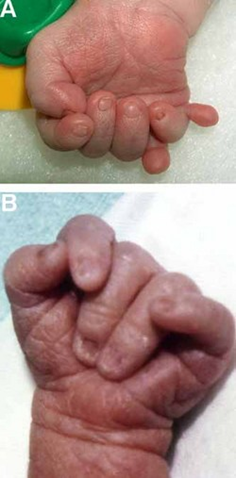
When the chromosomal abnormality is on pair #23, the result is a sex-linked chromosomal abnormality. A person might have XXY, XYY, XXX, XO, or 45 or 47 chromosomes as a result. Two of the more common sex-linked chromosomal disorders are Turner syndrome and Klinefelter syndrome. Turner’s syndrome occurs in 1 of every 2,500 live female births (Carroll, 2007) when an ovum which lacks a chromosome is fertilized by a sperm with an X chromosome. The resulting zygote has an XO composition. Fertilization by a Y sperm is not viable. Turner syndrome affects cognitive functioning and sexual maturation. The external genitalia appear normal, but breasts and ovaries do not develop fully and the woman does not menstruate. Turner’s syndrome also results in short stature and other physical characteristics. Klinefelter syndrome (XXY) occurs in 1 out of 700 live male births and results when an ovum containing an extra X chromosome is fertilized by a Y sperm. The Y chromosome stimulates the growth of male genitalia, but the additional X chromosome inhibits this development. An individual with Klinefelter syndrome has some breast development, infertility (this is the most common cause of infertility in males), and has low levels of testosterone.[37] [38] [39]
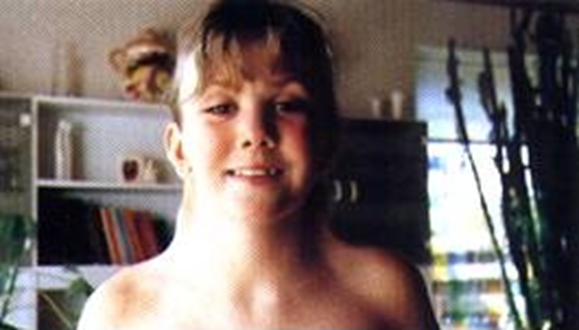
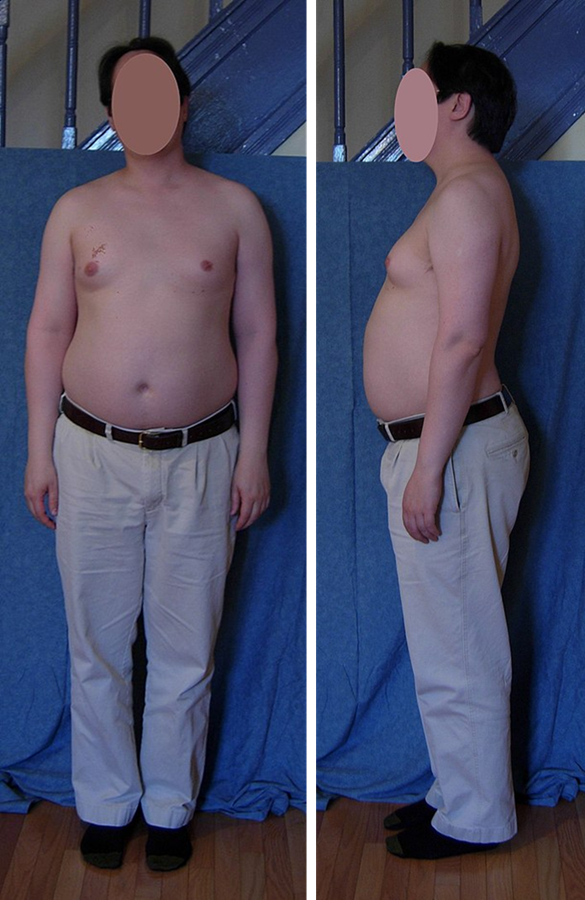
Genetic Counseling is a service that assists individuals identify, test for, and explain potential genetic conditions that could adversely affect themselves or their offspring is referred to as genetic counseling (CDC, 2015b). Some of the more common reasons for genetic counseling include:
- Family history of a genetic condition
- Membership in a certain ethnic group with a higher risk of a genetic condition
- Information regarding the results of genetic testing, including blood tests, amniocentesis, or ultra-sound
- Learning about the chances of having a baby with a genetic condition if the parents are older, have had several miscarriages, have offspring with birth defects, experience infertility, or have a medical condition[40]
Behavioral Genetics
Behavioral Genetics is the scientific study of the interplay between the genetic and environmental contributions to behavior. Often referred to as the nature/nurture debate, Gottlieb (1998, 2000, 2002) suggests an analytic framework for this debate that recognizes the interplay between the environment, behavior, and genetic expression. This bidirectional interplay suggests that the environment can affect the expression of genes just as genetic predispositions can impact a person’s potentials. Additionally, environmental circumstances can trigger symptoms of a genetic disorder. For example, a person who has sickle cell anemia, a recessive gene linked disorder, can experience a sickle cell crisis under conditions of oxygen deprivation. Someone predisposed genetically for type-two diabetes can trigger the disease through poor diet and little exercise.
Research has shown how the environment and genotype interact in several ways. Genotype-Environment Correlations refer to the processes by which genetic factors contribute to variations in the environment (Plomin, DeFries, Knopik, & Niederhiser, 2013). There are three types of genotype-environment correlations:
Passive genotype-environment correlation occurs when children passively inherit the genes and the environments their family provides. Certain behavioral characteristics, such as being athletically inclined, may run in families. The children have inherited both the genes that would enable success at these activities and given the environmental encouragement to engage in these actions.
Evocative genotype-environment correlation refers to how the social environment reacts to individuals based on their inherited characteristics. For example, whether one has a more outgoing or shy temperament will affect how he or she is treated by others.
Active genotype-environment correlation occurs when individuals seek out environments that support their genetic tendencies. This is also referred to as niche picking. For example, children who are musically inclined seek out music instruction and opportunities that facilitate their natural musical ability.
Genotype-Environment Interactions involve genetic susceptibility to the environment. Adoption studies provide evidence for genotype-environment interactions. For example, the Early Growth and Development Study (Leve, Neiderhiser, Scaramella, & Reiss, 2010) followed 360 adopted children and their adopted and biological parents in a longitudinal study. Results have shown that children whose biological parents exhibited psychopathology, exhibited significantly fewer behavior problems when their adoptive parents used more structured parenting than unstructured. Additionally, elevated psychopathology in adoptive parents increased the risk for the children’s development of behavior problems, but only when the biological parents’ psychopathology was high. Consequently, the results show how environmental effects on behavior differ based on the genotype, especially stressful environments on genetically at-risk children.
Lastly, epigenetics studies modifications in DNA that affect gene expression and are passed on when the cells divide. Environmental factors, such as nutrition, stress, and teratogens are thought to change gene expression by switching genes on and off. These gene changes can then be inherited by daughter cells. This would explain why monozygotic or identical twins may increasingly differ in gene expression with age. For example, Fraga et al. (2005) found that when examining differences in DNA, a group of monozygotic twins were indistinguishable during the early years. However, when the twins were older there were significant discrepancies in their gene expression, most likely due to different experiences. These differences included susceptibilities to disease and a range of personal characteristics.[41]
- Lifespan Development by Lumen Learning is licensed under CC BY 4.0 ↵
- Britannica, T. Editors of Encyclopedia (2023, March 30). Mitosis. Encyclopedia Britannica. Used under permissions given for educational purposes. ↵
- Biology 2e Open Stax. Senior Contributing Authors: Mary Ann Clark, Matthew Douglas, Jung Choi. Licensed under CC BY 4.0 ↵
- Children’s Development by Ana R. Leon is licensed under CC BY 4.0 ↵
- Anatomy &Physiology by OpenStax is licensed under CC BY 4.0 ↵
- Anatomy &Physiology by OpenStax is licensed under CC BY 4.0 ↵
- Anatomy &Physiology by OpenStax is licensed under CC BY 4.0 ↵
- Anatomy &Physiology by OpenStax is licensed under CC BY 4.0 (modified by Maria Pagano) ↵
- Anatomy &Physiology by OpenStax is licensed under CC BY 4.0 (modified by Maria Pagano) ↵
- Anatomy &Physiology by OpenStax is licensed under CC BY 4.0 (modified by Maria Pagano) ↵
- Anatomy &Physiology by OpenStax is licensed under CC BY 4.0 (modified by Maria Pagano) ↵
- Children’s Development by Ana R. Leon is licensed under CC BY 4.0 ↵
- Anatomy &Physiology by OpenStax is licensed under CC BY 4.0 ↵
- Lifespan Development: A Psychological Perspective by Martha Lally and Suzanne Valentine-French is licensed under CC BY-NC-SA 3.0 ↵
- Anatomy &Physiology by OpenStax is licensed under CC BY 4.0 (modified by Maria Pagano) ↵
- Anatomy &Physiology by OpenStax is licensed under CC BY 4.0 ↵
- Anatomy &Physiology by OpenStax is licensed under CC BY 4.0 ↵
- Children’s Development by Ana R. Leon is licensed under CC BY 4.0 (modified by Maria Pagano) ↵
- Lifespan Development: A Psychological Perspective by Martha Lally and Suzanne Valentine-French is licensed under CC BY-NC-SA 3.0 ↵
- Children’s Development by Ana R. Leon is licensed under CC BY 4.0 ↵
- Anatomy &Physiology by OpenStax is licensed under CC BY 4.0 ↵
- null ↵
- Text and Image from U.S Department of Health and Human Services, National Institutes of Health, National Cancer Institute found at https://www.cancer.gov/publications/dictionaries/genetics-dictionary/def/x-linked-recessive-inheritance Licensed under Public Domain ↵
- Text and image from Lumen Learning. Biology for Majors I. Licensed under CC BY 4.0 ↵
- Text and image from Lumen Learning. Biology for Majors I. Licensed under CC BY 4.0 ↵
- Text from Lumen Learning. Biology for Majors I. Licensed under CC BY 4.0 ↵
- From 12.2F: Lethal Inheritance Patterns is shared under a CC BY-SA 4.0 license and was authored, remixed, and/or curated by Boundless. ↵
- From NIH National Library of Medicine, National Center for Biotechnology Information. Loss of function mutations in essential genes cause embryonic lethality in pigs, by Martijn F. L. Derks, Arne B. Gjuvsland, Mirte Bosse, Marcos S. Lopes, Maren van Son, Barbara Harlizius, Beatrice F. Tan, Hanne Hamland, Eli Grindflek, Martien A. M. Groenen, and Hendrik-Jan Megens. Licensed under CC BY 4.0. Edited by Maria Pagano ↵
- From 12.2F: Lethal Inheritance Patterns is shared under a CC BY-SA 4.0 license and was authored, remixed, and/or curated by Boundless. ↵
- From Biology for Majors I. Module 9: DNA Structure and Replication by Lumen Learning. What is a Mutation by Genome Research Limited. Licensed under CC BY SA 4.0. ↵
- From Lumen Learning: Lifespan Development: Module 3: Prenatal Development. Licensed under CC BY 4.0. ↵
- Image from Wikimedia Commons. Child with Down Syndrome by NadineLive. Licensed under CC BY SA 4.0. ↵
- Text from NIH National Library of Medicine, Medline Plus. Trisomy 13. Licensed under Public Domain. ↵
- Illustration of Child with cleft lip and a child with cleft palate, from Centers for Disease Control and Prevention. Licensed under Public Domain. ↵
- Text from NIH National Library of Medicine, Medline Plus. Trisomy 18. Licensed under Public Domain. ↵
- Photograph of overlapping fingers from NIH Human Genome Research Institute. Licensed under Public Domain. ↵
- From Lumen Learning: Lifespan Development: Module 3: Prenatal Development. Licensed under CC BY 4.0. ↵
- Image of man with Klinefelter syndrome from Wikipedia, by Malcolm Gin. Licensed under CC BY SA 3.0. ↵
- Image Turner Syndrome webbed neck from Wikimekia Commons, by Johannes Nielsen. Licensed under CC BY 2.0. ↵
- Lifespan Development: A Psychological Perspective by Martha Lally and Suzanne Valentine-French is licensed under CC BY-NC-SA 3.0 (modified by Maria Pagano) ↵
- Lifespan Development: A Psychological Perspective by Martha Lally and Suzanne Valentine-French is licensed under CC BY-NC-SA 3.0 (modified by Maria Pagano) ↵
the international, collaborative research program whose goal was the complete mapping and understanding of all the genes of human beings. The publication was completed and published in October 2004.
the microscopic threadlike part of the cell that carries hereditary information in the form of genes.
a type of cell division that results in two daughter cells each having the same number and kind of chromosomes as the parent nucleus, typical of ordinary tissue growth.
prophase, the initial stage of mitosis and of the mitotic division of meiosis, characterized by the formation of the mitotic spindle and the condensation of the chromosomes. Prophase is followed by metaphase.
spherical body within the nucleus of most eukaryotic cells, involved in the synthesis of ribosomal RNA (rRNA) and the formation of ribosomes. Nucleoli appear shortly after mitosis and form around specific repeating chromosome regions, known as nucleolar organizing regions, within the nucleus. A single nucleus can house one to several nucleoli, depending on the organism and cell type.
metaphase, in mitosis and meiosis, the stage of cell division characterized by the alignment of the chromosomes along the midline of the cell. Metaphase is preceded by prophase and is followed by anaphase.
anaphase, in mitosis and meiosis, the stage of cell division in which separated chromatids (or homologous [like] chromosome pairs, as in the first meiotic division) move toward the opposite poles of the spindle apparatus. Anaphase is preceded by metaphase, in which the chromosomes line up along the midline of the cell, and is followed by telophase, which is the final step before the cell splits into two daughter cells.
one of a pair of daughter strands of a replicated chromosome. Chromatids serve an essential role in cell division, ensuring the accurate division and distribution of chromosomes to new daughter cells.
telophase, in mitosis and meiosis, the final stage of cell division in which the spindle disappears and the nucleus forms around each set of daughter chromosomes. Preceded by anaphase, telophase is usually followed by cytokinesis, in which the cytoplasm is physically divided to form two daughter cells. In meiosis, telophase I is followed by prophase II.
the semifluid substance of a cell that is external to the nuclear membrane and internal to the cellular membrane, sometimes described as the nonnuclear content of protoplasm.
a reproductive cell of an animal or plant. In animals, female gametes are called ova or egg cells, and male gametes are called sperm. Ova and sperm are haploid cells, with each cell carrying only one copy of each chromosome.
a type of cell division that results in four daughter cells each with half the number of chromosomes of the parent cell, as in the production of gametes
one of the numbered chromosomes, as opposed to the sex chromosomes. Humans have 22 pairs of autosomes and one pair of sex chromosomes (XX or XY)
the genetic makeup of an organism; the term can also be used to refer to alleles or variant forms of a gene.
an individual's observable traits, such as eye color
Two chromosomes in a pair – normally one inherited from the mother and one from the father
one of two or more alternative forms of a gene that are found at the same place on a chromosome.
having two identical alleles of a particular gene or genes.
having two different alleles of a particular gene or genes.
a table in which all of the possible outcomes for a genetic cross between two individuals with known genotypes is presented.
containing two complete sets of chromosomes, one from each parent.
inheritance of only one gene will produce the inherited trait.
a genetic condition occurs when the child inherits one mutated copy of a gene from each parent.
an individual who “carries” and can pass on a genomic variant (allele) associated with a disease or trait.
caused by variants in genes on the X chromosome
genetic conditions associated with mutations in genes on the X chromosome. A single copy of the mutation is enough to cause the disease in both males (who have one X chromosome) and females (who have two X chromosomes).
results from a cross in which each parental contribution is genetically unique and gives rise to progeny whose phenotype is intermediate.
a type of inheritance in which two versions (alleles) of the same gene are expressed separately to yield different traits in an individual.
a blood group system that is determined by the presence or absence of two antigens, M and N, on the red blood cells. The MN blood group system is a minor blood group system.
a rare blood type where both A and B antigens on the surface of red blood cells are found, but no antibodies are found in the plasma.
a condition that damages nerve cells in the brain causing them to stop working properly, which can affect movement, cognition (perception, awareness, thinking, judgement) and mental health.
in this case genetic mutations are changes to your DNA sequence that happen during cell division when your cells make copies of themselves.
a genetic disorder caused when abnormal cell division results in an extra full or partial copy of chromosome 21.
a clinical syndrome that occurs when all or some cells of the body contain an extra copy of chromosome 13.
a birth defect in which one or both eyes did not develop fully, so they are small.
occurs when the tissue that makes up the lip does not join completely before birth.
occurs when the tissue that makes up the roof of the mouth does not join together completely during pregnancy.
a disorder in which babies are born with 3 copies of chromosome 18 instead of 2. For an unknown reason, this accident occurs at the time of conception and all cells in the body will have this structure
a condition that affects only females, results when one of the X chromosomes (sex chromosomes) is missing or partially missing. Turner syndrome can cause a variety of medical and developmental problems, including short height, failure of the ovaries to develop and heart defects.
a common genetic condition where a male is born with an extra X chromosome. People with Klinefelter syndrome can experience breast growth, breast cancer, osteoporosis, infertility and learning difficulties.
the study of genetic and environmental influences on behaviors.
the association between an individual's genetically influenced behavior and others' reactions to that behavior.
the association between the genotype a child inherits from his or her parents and the environment in which the child is raised.
the association between an individual's genetically influenced behavior and others' reactions to that behavior.
the association between an individual's genetic propensities and the environmental niches that individual selects.
the interplay of genes (and, more broadly, genome function) and the physical and social environment. These interactions influence the expression of phenotypes. For example, most human traits and diseases are influenced by how one or more genes interact in complex ways with environmental factors, such as chemicals in the air or water.
the study of how your behaviors and environment can cause changes that affect the way your genes work.